Nature as the Source of Materials for More Sustainable Organic Electronics
Yasser Matos-Peralta, Manuel Reali, Anthony Camus, Dieudonné Niyonkuru, Molood Hoseinsizadeh, Zhaojing Gao, Clara Santato*
Engineering Physics, Polytechnique Montréal, 2900 Boulevard Edouard Montpetit, Montréal, Québec, H3T 1J4, Canada.
Introduction
The need for eco-friendly technologies is the underpinning of Sustainable Organic Electronics (SOE) and their powering elements.1 SOE uses carbon-based electronically conjugated (i.e., featuring alternance of single and double carbon-carbon bonds) molecular materials extracted from bio-sourced sources or synthesized along the principles of “green” chemistry.2,3 To limit the device embodied energy (i.e., the energy associated with device manufacturing, including energy for extracting, refining, processing, and transporting primary materials), materials for SOE are usually solution-processed (e.g., printed electronics). SOE is meant to limit the use of critical chemical elements used in conventional electronics.4,5 Further, organic electronic materials and devices can be eco-designed for biodegradation at their end-of-life, thus helping alleviate the accumulation of Waste of Electrical and Electronic Equipment (WEEE or e-waste, summing up to 53.6 Mt at the global level in 2019, equivalent to 4500 Eiffel towers).1,5
Indigoids, melanins, chlorophylls, and tannins are examples of bio-sourced materials under investigation in SOE6–8 (Figure 1, where DHI and DHICA are the building blocks of eumelanin — the black-brown form of melanin — catechin and tannic acid belong to the tannin family of molecules). For instance, tannins can be extracted from forest residues and agricultural biomass waste. They can also be obtained as by-products of the food industry.
In organic materials, the weak van der Waals intermolecular interactions (ca. 0.4-4 kJ/mol, 4 to 40 meV per chemical bond) cause structural and energetic disorder that affect the mechanism of electronic transport in the materials and, consequently, the performance of devices based thereon. Structural disorder can be caused by the twist of molecular backbones, longer (or shorter)-than-average conjugation lengths, molecular chains not arranged in periodic structures, or the existence of π-π stacked regions with different extensions and orientations.9 Today, various transport models are proposed, but they have certain limitations and a universal model has not yet been developed.10

Figure 1.Molecular structures of interest in SOE: chlorophyll a, tannic acid, 5,6-dihydroxyindole (DHI), 5,6-dihydroxyindole- 2-carboxylic acid (DHICA), catechin, and indigo.
Challenges With Bio-sourced Organic Semiconductors
Charge carriers in organic semiconductors (OSCs) feature lower mobility (typically 10−1–10−5 cm2 V−1 s−1) than their inorganic counterparts.11 Most natural and synthetic OSCs suffer from high structural disorder because of weak intermolecular van der Waals interactions holding the molecules together in films amenable to devices. This causes short-range-electron delocalization, a limiting factor for electronic transport.12 Open challenges in the OSCs field are their poor environmental (shelf life) operational stability and limited multifunctionality, e.g., the possibility to combine efficient transport and electroluminescence as needed in organic light-emitting organic transistors.
Concerning device stability, Organic Field Effect Transistors (OFETs) based on hydrogen-bonded air-stable organic semiconductors, such as indigo and Tyrian purple, reached mobility in the range of 0.01–2 cm2 V-1 s-1.4,13 Quinones are small organic molecules ubiquitous in nature that contain two adjacent (or separated) carbonyl groups in an unsaturated six-carbon ring structure; quinone derivatives (i.e., anthraquinones AQ, benzoquinones BQ, N-heteropentacenequinones HAQ, quinacridones QA) are among the most interesting hydrogen-bonded semiconductors.4
Owing to the structural disorder of bio-sourced materials of interest in organic electronics, it is difficult to know the position of their HOMO and LUMO energy levels since the structural disorder implies energetic disorder. Only very recently, studies by ultraviolet photoemission spectroscopy (UPS) and inverse photoemission spectroscopy (IPES) gave insight into the LUMO and HOMO levels of building blocks of the biopigment eumelanin, i.e., 5,6-dihydroxyindole of (DHI) and 5,6-dihydroxyindole-2-carboxylic acid (DHICA).14 The need to understand the energy levels poses a challenge to engineering efficient metal electrode/organic material device interfaces, for example, when minimizing the charge carrier injection (Schottky) barrier. The Schottky barrier is the energy offset between the Fermi level of the metal electrodes and the HOMO and LUMO levels of the material.15 One-dimensional carbon nanotube (CNT)-based electrodes offer the opportunity of efficient charge carrier injection due to favorable electrostatic effects, which permits circumvention of injection barriers.15,16
Powering Elements for SOE: Energy Conversion and Storage
Quinone-based materials are promising for applications in renewable energy, such as solar energy conversion and electrochemical energy storage.7 Quinones feature varying redox states: fully oxidized quinones, semiquinones, and reduced hydroquinones. In aqueous solutions, quinones undergo two-electron, proton-coupled electron transfers. Furthermore, quinones show high metal ion binding affinity, e.g., through chelation and/or electrostatic interactions. Such affinity is relevant in electrochemical energy storage devices that rely on mixed ionic and electronic transport, such as batteries and supercapacitors.7 It is worth noting that the metal ion binding affinity also permits metal recovery and separation for water purification and e-waste treatment applications by biohydrometallurgy techniques.17 Other properties of quinones include antioxidant activity and medicinal chemistry.18
Quinones-based biopigments, such as melanins and tannins, have been applied in batteries and electrochemical capacitors (supercapacitors). We have reported on supercapacitors based on carbon paper electrodes, surface-modified by solution-processed melanins or tannins.19,20 To tackle challenges such as poor electronic coupling between the quinone-based material and the current collectors and low cycling stability (i.e., loss of capacitance over long charging/discharging cycling), we chemically treated the carbon paper in a way to improve electrolyte wettability, enhance the surface area, and tailor surface porosity.20 Quinones in electrochemical systems can feature their poor electrical conductivity. Here, the supramolecular organization plays a vital role in the quinone-based materials. Theoretical studies can guide the engineering of such organization.21,22 Furthermore, to limit the release of the quinones by solubilization into electrolytes, it is helpful to use semi-solid-state electrolytes such as ion gels that also bring mechanical integrity to the devices. Semi-solid-state electrolytes that feature ionic mobility and mechanical flexibility are a combination of polymers such as poly(ethyl oxide) (PEO, like Cat. Nos. 372781, 182028, 372773) or poly(vinyl alcohol) (PVA, Cat. No. 360627), with salts such as NaCl, MgCl2, and Na2SO4.23
Quinones-based materials can feature high UV-Vis absorption, suitable for light-assisted electrochemical energy storage and solar energy conversion applications, e.g., dye-sensitized photoelectrochemical cells.7,21,24 (See Figure 2).
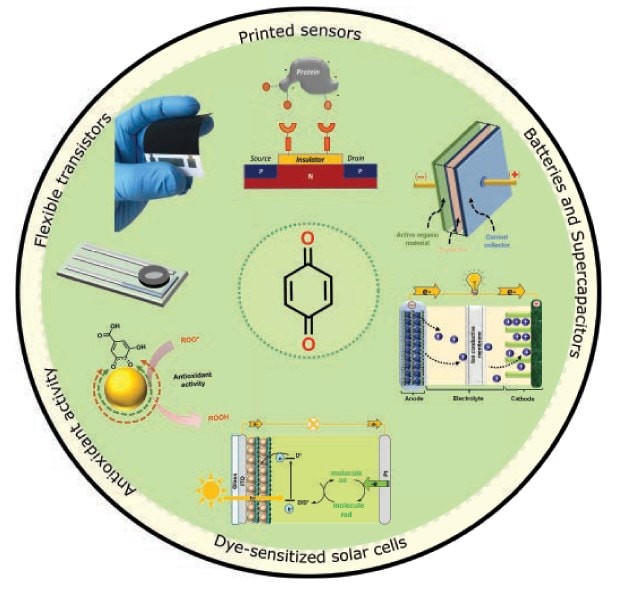
Figure 2.Technological applications making use of quinone-based materials.
Printing
The fabrication of films and devices through printing is of primary importance in developing sustainable organic electronic devices. Printing is an additive process that offers the possibility to i) reduce the waste of materials,25 ii) reduce the energy required to fabricate a device,26 iii) reduce the overall manufacturing cost, and iv) fabricate flexible devices. Moreover, printing is a scalable technology that can provide a high manufacturing throughput (e.g., gravure printing and flexography up to 102 m2 s-1).27
Among various industrial printing technologies, gravure printing, reverse offset, and inkjet printing are promising in terms of scalability, printing speed, and spatial resolution. Printing technologies are rapidly evolving and can achieve printed feature sizes down to a few microns (as well as down to sub-microns).25,28
The formulation of inks consists of combining semiconducting (e.g., Cat. Nos. 923184, 923222, 924385, 923230, 923192, 902012, 900958, 906980) or conducting (e.g., Cat. Nos. 923567, 923575, 923559, 900208, 900181, 793663, 900695, 900960) active materials with solution processable polymer binders. Blended inks are two-phase systems (polymer matrix/active material), possibly forming percolating networks for charge carriers.29
Bio-sourced materials (extracted from natural sources) can benefit from printing technologies. Insulating natural polymers like Shellac (Kerria lacca) can be used as a biodegradable binding agent to formulate ink blends.29 Nanostructured bio-sourced materials featuring compelling charge carrier transport properties but poor solution processability, such as Sepia melanin (e.g., extracted from the ink sac of Sepia officinalis), could take advantage of the protecting and stabilizing properties of binders.30
It is worth mentioning that one of the biggest challenges in printing technologies is related to the sustainability of the solvents used. Many of the utilized solvents are based on toxic volatile organic compounds (VOCs) that are environmental pollutants. Therefore, it is necessary to search for more ecological solvent solutions. However, substituting a solvent for an eco-friendlier one is a challenging task. It should be considered that the new solvent must dissolve the solutes and allow adequate ink-substrate wetting, ink-film formation, and correct drying of solute-film during the printing/coating process.31 Generally, water is the ideal greener solvent to use in this process. Still, it is necessary to consider solute affinity because various organic molecules and polymers are not soluble in water. Other greener solvents to consider are alcohols and mixtures of alcohols (e.g., 1-propanol, isopropanol Cat. No. 190764), anisole (Cat. No. 296295), ethoxybenzene, and N,N′-dimethylpropyleneurea.32
Biodegradation
Biodegradation of devices at their end-of-life is one of the critical challenges in developing sustainable organic electronic devices. We wish to point out that being organic, bio-sourced does not mean automatically to be biodegradable.33,34 When biodegradation happens in compost conditions (it is worth mentioning that biodegradable organic devices have also been investigated for biomedical technologies35), the molecular and supramolecular structures of some bio-sourced organic molecules and polymers can cause recalcitrance to microbial attack during the degradation. Aspects such as humidity, pH, temperature, and solar illumination must be considered to gain insight into factors promoting or inhibiting biodegradation.2,17,34 It is worth noting the possible (eco)toxicity of chemical intermediates in the biodegradation process (i.e., the biodegradation process should be continuously monitored to characterize chemical intermediates). Sustainable organic electronics is a research area still in its infancy, such that biodegradability tests are not yet standardized. Compostability tests for conventional (insulating) plastics are CAN/BNQ 0017-088/2010, ASTM D6400, EN 13432, and EN 14995.17,33 As an example of biodegradability study for sustainable organic electronics, our study related to the biodegradation of eumelanin in mesophilic and thermophilic conditions employed the ASTM D5338 standard test for the aerobic biodegradation of plastic materials under controlled composting conditions.33
Conclusions and Perspectives
The dramatic impact of electronics on the environment and human health requires a paradigm shift from linear to circular electronics. Here, we indicate responsible and respectful use of resources, responsible manufacturing practices, and practical electronic end-of-life management. To realize such a shift, eco-design of electronics and extended producer responsibility should be at the core of all related efforts.
We believe several responses are needed to develop sustainable electronics tailored to consumers’ needs and local constraints. For efficient composting, engineering of the microbiota should be pursued due to possible traces of device metal contacts acting as biodegradation inhibiting agents. Additionally, the conductivity of carbon-based contacts is usually lower than that of conventional metals-based counterparts (e.g., Au). Disassemblable (modular) devices, wherein metal contact-patterned substrates can be reused, could offer a viable solution.
As in other industrial sectors, waste should be a keyword. E-waste is an opportunity, such as the recovery of precious metals in the recycling process. The concept of waste should evolve; it should be seen as a step in the life of materials to be used to produce new materials. Organic electronic materials offer a possibility to alleviate the impact of electronics on the environment and human health for several reasons: they are based on carbon, an abundant element, and they can be printed for low-embodied-energy devices. End-of-life scenarios for these materials, such as composting, can easily be foreseen.
References
Per continuare a leggere, autenticati o crea un account.
Non hai un Account?