Poly(ethylene glycol) (PEG) and Synthetic PEG Derivatives for Tissue Engineering and Cell Delivery
Ali Affar, Fei Xu, Todd Hoare
McMaster University, Department of Chemical Engineering, 1280 Main Street West, Hamilton, Ontario, L8S 4L8, Canada.
Material Matters, 2018, 13.3
Introduction
Tissue engineering and regenerative medicine offers immense promise for improving and prolonging life, with several cell-based therapies now starting to reach the clinic. From functionally replacing entire organs to delivering therapeutic cells to an injured site, the success of any tissue engineering strategy is dependent on the biomaterial scaffold used to deliver and/or develop cells both in vitro and in vivo.1 Successful tissue engineering scaffolds have several properties in common: (1) they must be reproducibly fabricated (part of the attractiveness of 3D printing strategies);2 (2) they must be easy to deliver to the desired site of action, preferably via injection;1,3 (3) they should ideally match the physical and mechanical properties of the extracellular matrix (ECM) of the native tissue;4 (4) they must support the desired cell functionality, including adhesion, proliferation and/or differentiation;5 (5) they should induce minimal or no inflammatory response; and (6) they must degrade at an optimal rate into nontoxic byproducts that are generally regarded as safe for clearance by the body.
Hydrogels, are water-soluble polymer networks that are well-suited as tissue engineering scaffolds given their high water content, typically low inflammatory responses, tunable biomimetic mechanics, and highly variable chemistries. In particular, a wide variety of naturally occurring biopolymers such as polysaccharides, proteins, or nucleic acids (many of which can be found in the native ECM of cells) have been explored for use in tissue engineering applications.6 Biopolymers generally exhibit high cytocompatibility and can often degrade into safe extracellular matrix components like sugars and amino acids. However, the inherent bioactivity and the difficulty of reproducibly functionalizing such materials makes it difficult to control key properties such as degradation rate and mechanics. Synthetic polymers can address these limitations since they can be engineered to produce hydrogels with well-defined chemistries, structures, mechanics, and residual functionalities. In particular, a synthetic approach allows for the incorporation of multiple functional moieties, including some that introduce “smart” properties such as in situ gelation, thermosensitivity, or pH-responsiveness, via typically facile copolymerization strategies.4,7 In some cases, synthetic polymers can also be tailored to degrade into small molecules; for example, the degradable polymer poly(lactide-co-glycolide) (PLGA) can degrade into the natural metabolites lactic acid and glycolic acid. However, there are fewer examples of water-soluble hydrogel precursor polymers with such functionality.4 Alternately, synthetic hydrogels can be developed to enable clearance by the renal system by controlling the polymer size and polydispersity and linking the polymers with degradable crosslinks.3
Among all reported synthetic polymers, poly (ethylene glycol) (PEG) has been the most extensively studied for tissue engineering. PEG offers the benefits of a hydrated structure, resulting in typically high cytocompatibility and protein repellency to minimize inflammatory responses.8 While the hydrophilicity of PEG makes it generally non-adhesive to cells, this can be overcome by incorporating cellular adhesive motifs such as arginylglycylaspartic acid (RGD) within the hydrogel.7 Based on these properties, PEG-based hydrogels have been successfully applied to a range of tissue engineering and cell delivery applications.9 However conventional PEG-based hydrogels are limited in terms of their lack of injectability, lack of tunable degradation, and relatively poor mechanics. Herein, we describe how changing the crosslinking chemistry and/or the chain structure of the PEG starting materials can improve the performance of PEG and PEG-derivative hydrogels for tissue engineering or cell delivery applications.
Crosslinking chemistry
Free radical polymerization of poly(ethylene glycol) diacrylates is the most common strategy for preparing PEG-based hydrogels, with the degree of crosslinking adjusted by changing the length of the PEG chain between the acrylate groups.10 However, the low swelling ratio, non-degradability, lack of injectability, and uncontrolled mesh size of the resulting hydrogels can create limitations in biomedical applications. Alternative crosslinking strategies have thus been designed to facilitate both injectability and degradability in PEG-based networks.
Injectability
To create injectable PEG-based hydrogels, the most common strategy is to co-extrude two PEG derivatives functionalized with complementary groups that can physically or chemically crosslink. Covalent in situ-gelation, the more common of the two in tissue engineering, is enabled by converting the hydroxyl end group of PEG to a variety of rapidly reactive functional groups to enable one or more of the various in situ crosslinking chemistries shown in Figure 1. By controlling the number, length, and reversibility of crosslinks formed, the physical properties of a hydrogel can be tuned.3

Figure 1.Examples of different in situ crosslinking chemistries: A) Michael addition; B) disulfide bond formation; C) hydrazone condensation; D) oxime formation; E) Diels-Alder cycloaddition; F) strain-promoted alkyne-azide Huisgen cycloaddition. (Reproduced with permission from reference 3, copyright 2014 John Wiley & Sons).
While all these chemistries have been applied successfully to various cell scaffolding applications, each has distinct advantages and drawbacks. Michael addition chemistry (Figure 1A) is relatively fast but creates a non-degradable linkage and can interact with proteins in the body. Disulfide formation (Figure 1B) yields redox-responsive hydrogels but is typically slower and yields weaker gels. Hydrazone chemistry (Figure 1C) is rapid and forms a hydrolyzable hydrazone bond but typically uses aldehyde groups that can form Schiff bases with amines in proteins. Oxime chemistry (Figure 1D) forms a more slowly degradable bond appropriate for longer-lasting scaffolds but requires acid catalysis for rapid gelation. Diels-Alder chemistry (Figure 1E) is highly bio-orthogonal but gels somewhat slowly and creates functionally irreversible crosslinks (at least without high temperatures). Strain-promoted alkyne/azide cycloaddition (Figure 1F) is rapid and highly specific but introduces significantly hydrophobicity into the hydrogel. Thus, the crosslinking chemistry must be chosen carefully in terms of balancing the gelation rate, the potential for side-reactions, and the rate of degradation most appropriate for each application.
Non-covalent interactions such as electrostatics, hydrogen bonding, stereocomplexation, or hydrophobic interactions can also be used to facilitate in situ gelation or create highly shear-thinning hydrogels that still enable injection.11 However, these strategies often lead to hydrogels with poor stability in the highly diluted environment of the body and/or are subject to interferences in vivo that disrupt crosslinking. Inclusion complexes prepared using cyclic supramolecular structures like alpha-cyclodextrin (CD) as a host for polymers such as poly (ethylene glycol) do offer an interesting alternative though to covalent crosslinking.12 Multiple PEG chains can thread through the center of the CD and interact via hydrogen bonding/dipole interactions to form a crosslinking point. Of particular relevance to tissue engineering, the mobility of the inclusion complex crosslinks (unlike covalent crosslinks) can lead to selfhealing hydrogel structures. Combinations of covalent and noncovalent crosslinking strategies thus offer intriguing options that can address the drawbacks of both covalent and physical approaches. For example, Qiao et al. functionalized PEG with alkyne end-groups and CD rings with reactive azide groups to enabling both non-covalent (CD-PEG interactions) and covalent (alkyne/azide click chemistry) crosslinking (Figure 2). Both HeLa and HEK293T cells could be maintained inside the scaffold with greater than 90% viability over 10 days, and the gel can undergo complete degradation within 1 month.13

Figure 2.A schematic of the different network formation strategies applied in a hydrogel produced from alkyne-functionalized PEG and azide-functionalized CD. (Reproduced with permission from reference 13, copyright 2013 the Royal Society of Chemistry)
Degradability
To enable optimal tissue regeneration, the degradation rate of a scaffold should match the rate at which the cells inside propagate through the matrix and generate their own extracellular matrix, recreating native biology. Although common degradation mechanisms including hydrolysis may be engineered to enable such control, the use of specific stimuli such as light or disease state to dynamically degrade the gel under specific environmental conditions in which degradation is desirable is highly attractive.5
Enzymatic degradation is the most popular of these approaches, in which specific amino acid sequences that are substrates to various enzymes (including those specifically up-regulated in disease) are used as crosslinkers. The most commonly reported substrate is for matrix metalloproteases (MMPs), cell-secreted enzymes overexpressed in several inflammatory conditions and cancers.7 For example, Bryant et al. reported a PEG-based hydrogel loaded with pre-osteoblast cells and hydroxyapatite nanoparticles and crosslinked with MMP-sensitive peptide sequences; upon upregulation of MMPs during osteogenesis, the degradation rate of the hydrogel was accelerated to promote bone regeneration.9
UV or visible light has also been used to induce targeted degradation in hydrogels. Crosslinks can be manipulated by either light-induced cis-trans isomerization (e.g. azobenzenes or stilbens) or reversible ring-opening/ring-closing isomerization (e.g. diarylethenes, spiropyrans, fulgimides) (Figure 3).5 Although such an approach is largely limited to in vitro use due to the low penetration of light through the body, it can be used in in vivo topical, dental, and optical implants to trigger photoinduced degradation events to either degrade or decrease the stiffness of the gel, the latter useful for promoting cell proliferation and/or inducing specific cell differentiation. For example, Anseth’s group produced a cellularized hydrogel by crosslinking thiolated 4-armed star PEG and acrylatefunctionalized poly(ethylene glycol) di-photodegradable acrylate (PEGdiPDA) via Michael addition chemistry and subsequently degraded the hydrogel via cleavage of the o-nitrobenzyl ether photodegradable linker by UV irradiation.14 The on-demand switching of the hydrogel porosity and mechanics enabled precise control over cellular responses such as single stem cell migration15 and cell differentiation (e.g. the fibroblast-tomyofibroblast transition).16

Figure 3.Four examples of photoresponsive crosslinking approaches. (Reproduced with permission from referece 5, copyright 2017 the Royal Society of Chemistry)
Chain structure
The chemical structure of PEG and PEG-based derivatives plays an important role in regulating the hydrophilicity, biological responses, mechanical properties, protein repellency, degradation kinetics, and swelling of PEG-based hydrogels.17 Among the more common modifications made is to attach biodegradable hydrophobic polymer blocks based on polymers such as poly (lactic acid) (PLA), poly (lactide-co-glycolide) (PLGA), or poly(propylene oxide) (PPO) to PEG to form diblock or triblock copolymers that can self-associate into hydrogels via hydrophobic interactions but degrade over time as the hydrophobic polymer degrades.18 Triblock copolymers, particularly PEO-PPO-PEO (i.e. the Pluronics family of polymers), have been frequently investigated given their sol-gel transition behavior upon heating from room temperature to physiological temperature, which enables injection. For example, Kolesky et al. demonstrated the use of PEO-PPO-PEO as a thermogelling cell-laden ink to create vascularized tissue constructs containing fibroblast cells by bioprinting.19 The susceptibility of these networks to dilution over time as well as their relatively poor mechanics limit such materials to use in engineering lower modulus tissues, although combining such polymers with covalent bond forming units (e.g. using diacrylated Pluronic F-127)20 can in part address this drawback.
Changing the physical arrangement of the PEG chains from linear chains to more complex geometries also has significant impacts on gel properties. In particular, star or branched arrangements of PEG chains enable the formation of higher modulus and chemically tunable PEG hydrogels. This is due to the increasing number of crosslinkable groups per single chain compared to linear PEG, which has only one –OH group at each chain end.21 Spatially organizing PEG chains in the precursor materials also allows for the formation of more ordered and welldefined chemical structures, beneficial for promoting desirable cell responses.22
Star PEG polymers are typically prepared by connecting 4, 6 or 8 arms of linear PEG (of tunable length) to a single internal point; the end group of each arm can be functionalized with biomolecules or crosslinkers as desired to create gels with more tunable mechanics and biological activities.23 Indeed, tetrahedron-oriented 4-arm star PEGs have been shown to produce highly homogeneous network structures, enabling the production of hydrogels with moduli in the MPa range.24 Star-PEGs can also be used in conjunction with injectable/degradable chemistries to form tissue scaffolds. For example, amphiphilic 8-armed PEG-b-PLA-cholesterol copolymers thermally gel at 34 °C due to interactions between the cholesterol groups to create a microstructured network that can support L929 mouse fibroblast cell viability and proliferation in the hydrogel.25 Leveraging the additional surface functionality of star PEGs also allows for the creation of highly functional hydrogels without sacrificing crosslinking density. For example, RGD modified star PEG coatings can significantly enhance cell spreading compared to non-RGD functionalized star PEG or RGD-functionalized linear PEG gels given the higher density of RGD that can be grafted using a star-PEG morphology.26
To avoid the multiple-step synthesis of star PEGs, hyperbranched PEG copolymers (globular chains with molecular weights ranging from 1400 g/mol to 1,700,000 g/mol) have been synthesized based on random anionic ring-opening copolymerization of ethylene oxide in the presence of glycidol.27 This one-step free radical reaction method to create a PEG derivative with a high number of functionalizable chain ends is a synthetically and practically attractive, although it produces a more polydisperse starting unit than star PEGs (leading to weaker gels) and can result in low yields. To our knowledge, such materials have not yet been widely explored for tissue engineering applications.
Branched PEG copolymers offer an alternate and highly attractive option for creating PEG-based hydrogels. The most common branched PEG materials are based on poly(oligoethylene glycol methacrylate) (POEGMA), which consists of a methacrylate backbone and one PEG side chain of tunable length per monomer repeat unit.17 Such a structure offers facile polymerization via free or controlled radical processes enabling the formation of both linear and hyperbranched structures as well easy functionalizability by copolymerization.28 In addition, changing the length of the PEG side chain significantly changes the properties of the hydrogel. For example, Lutz and coworkers demonstrated that copolymerization of long side chain oligo (ethylene glycol) methyl ether methacrylate (OEGMA) and short side chain di(ethylene glycol) methyl ether methacrylate (M(EO)2MA) monomers leads to polymers and hydrogels with a precisely tunable lower critical solution temperature (LCST) between room temperature to > 80 °C according to the ratio of OEGMA and M(EO)2MA used.29 LCST behavior converts the hydrogel from highly hydrophilic to somewhat hydrophobic, driving significant changes in cell adhesion and thus tunable cell delamination (Figure 4).30
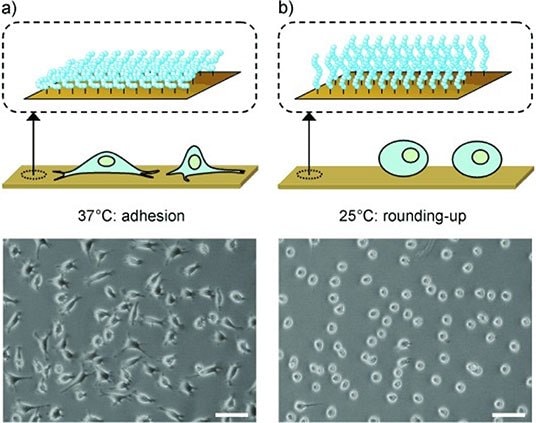
Figure 4.Microscopy images of L929 mouse fibroblasts on poly(OEGMAco-M(EO)2MA)-modified gold substrates after A) 44 hours at 37 °C and B) 25 °C for 30 mins. Scale bars = 100 mm. (Reproduced with permission from reference 30, copyright 2008 John Wiley and Sons).
Our lab has actively developed injectable or printable analogues of POEGMA hydrogels by functionalizing POEGMA precursor polymers with hydrazide and aldehyde reactive functional groups, enabling in situ gelation following mixingbased coextrusion via hydrazone crosslinking.31 The gelation time can be adjusted from minutes to hours for specific applications by changing the concentration, molecular weight, and/or density of reactive functional groups on the precursor polymers, enabling facile injection
in vivo as well as processing via techniques like electrospinning to create well-defined nanofibrous hydrogel networks with the potential to mimic the nanofibrous extracellular matrix32 or ink jet printing to create immobilized hydrogel coatings on porous supports.33 The PEG-based chemistry maintains low inflammatory responses upon injection in vivo, while the hydrazone gelation chemistry facilitates hydrolytic degradation over time; indeed, others have shown that branched PEGs can avoid some of the emerging immune responses observed with PEG-based materials.34 In addition, the facile copolymerizability of POEGMA enables us to create a variety of functional POEGMA-based copolymers that are injectable while also introducing the potential for hydrophobic domain formation, temperature responsive swelling, cell delamination, or cell-adhesive properties, among others (Figure 5).17

Figure 5.Library of functionalized hydrazide and aldehyde-functionalized poly(oligoethylene glycol methacrylate) (POEGMA) hydrogel precursors prepared via functional monomer copolymerization and/or post-polymerization grafting. (Reproduced with permission from reference 17, copyright 2015 Royal Society of Chemistry).
In particular, from a cell delivery context, the introduction of charged groups by copolymerizing POEGMA with acrylic acid (AA, anionic charge) and/or N,N-dimethylaminoethyl methacrylate (DMAEMA, cationic charge) demonstrated significantly enhanced cell adhesion, with amphoteric hydrogels prepared by mixing cationic and anionic-functionalized precursor polymers in particular demonstrating improved cell viability for long-term encapsulation and delivery of retinal pigment epithelial cells to the back of the eye (Figure 6).35
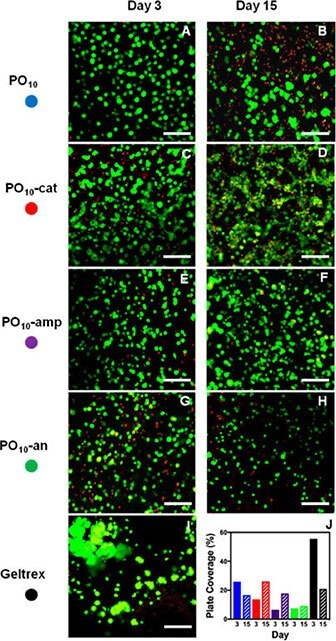
Figure 6.3D encapsulation of ARPE-19 retinal epithelial cells imaged using confocal microscopy after 3 days and 15 days in (A,B) uncharged, (C,D) cationic, (E,F) amphoteric, and (G,H) anionic POEGMA injectable hydrogels compared to (I) a Geltrex matrix control. The percentage of fluorescence plate coverage of live cells for each gel and time point is shown in (J). Scale bar = 100 μm. (Reproduced with permission from reference 35, copyright 2017 American Chemical Society).
Conclusions
While the bio-inert nature of PEG has allowed PEG hydrogels to be used effectively in a variety of tissue engineering and cell delivery applications, the tunable chemical properties of PEG derivatives such as injectable and star/branched PEGs offer unique potential to control the chemistry, degradability, injectability, and mechanics of PEG-based hydrogels to improve their performance in cell-based applications. In particular, we believe the significantly improved chemical flexibility of PEG derivative hydrogels can address the inherent challenges of conventional PEG hydrogels, particularly in terms of improving the mechanics and cell-hydrogel interactions to direct desired tissue growth or cell differentiation/maintenance responses.
References
To continue reading please sign in or create an account.
Don't Have An Account?