Synthesis, Properties, and Applications of Perovskite-Phase Metal Oxide Nanostructures
Yuanbing Mao1, Hongjun Zhou1, Stanislaus S. Wong1, 2
1Department of Chemistry, State University of New York at Stony Brook, Stony Brook, NY 11794-3400, 2Condensed Matter Physics and Materials Sciences Department, Brookhaven National Laboratory, Upton, NY 11973
Material Matters 2010, 5.2, 50.
Properties of Perovskite Systems
Perovskite-phase metal oxides exhibit a variety of interesting physical properties which include ferroelectric, dielectric, pyroelectric, and piezoelectric behavior.1-4 Specifically, linear dielectric materials exhibit linear polarization behavior as a function of applied field. Ferroelectric ceramics are dielectrics with a permanent electric dipole, which can be oriented upon the application of an electric field. Pyroelectric materials yield a spontaneous polarization, but the direction of such polarization cannot be reversed upon application of an electric field. Piezoelectrics either exhibit an electrical charge when mechanically stressed or undergo mechanical deformation upon the application of an electric field. In general, these various properties arise from the crystal symmetry adopted by these materials.5,6
Of these various classes of structures, ferroelectric materials are both pyroelectric and piezoelectric and are generating a great deal of current interest because their electric dipoles can be reoriented by the application of an electric field. However, all of these materials lose their inherent polar properties above their Curie temperature (Tc) transforming into a nonpolar state known as the paraelectric phase. For example, barium titanate (BaTiO3, Prod. No. 467634) alters from a tetragonal ferroelectric phase to a paraelectric cubic phase at a Curie temperature of 120 °C. The properties of ferroelectric materials change significantly and show a maximum as the temperature approaches Tc. Specifically, in the case of BaTiO3,7 the dielectric constant increases from 1,000 to a value of ~10,000 in the 10 K interval below its Tc, while above this temperature, there is a gradual decrease in the dielectric constant. Hence, the main applications of BaTiO3 are based around this phase transition, especially with respect to its potential as a dielectric. The temperatures at which these phase transitions occur can also be modified by the partial substitution of other metal oxides into BaTiO3 so as to yield solid solutions. For example, dopant substitutions can change cell dimensions and hence the temperatures at which these phase changes occur. Doped BaTiO3 has found wide applications8 in PTC (positive temperature coefficient) thermistors, semiconductors, and piezoelectric devices.
Different physical properties of perovskite-phase materials are related to their phase transitions, which in turn are sensitive to variables such as chemical composition, purity, number of surface and bulk defects, grain size, and sintering conditions. Hence, the need to control these parameters is critical for effective quality control of the devices produced from these materials. For instance, studies have been conducted on the effect of the crystallite size on the crystalline structure adopted by BaTiO3. As an example, crystals of BaTiO3 are often cubic at their surface but tetragonal in the bulk, with the two phases separated by a transition zone. As the crystallite size decreases below a few micrometers, the proportion of the crystal influenced by the surface effect increases and any undistorted tetragonal phase disappears. Thus, if the crystallite is small enough (nanosized), then the ferroelectric state disappears altogether and is replaced by a pseudocubic, superparaelectric state.9
Perovskite-type oxides with typically very high melting points also exhibit a number of interesting chemical properties including heterogeneous catalytic activity. For example, these oxides are often utilized for CO oxidation, oxidation of hydrocarbons and chlorinated volatile organic compounds, partial oxidation of methane to synthesis gas, N2O decomposition, NOx reduction, hydrogenation reactions of alkenes, SO2 reduction, as well as in various types of electro- and photocatalytic reactions.10,11
There is a variety of dense perovskite-phase mixed-metal oxides, which are capable of high oxygen transport fluxes at elevated temperatures (T >600 °C). At high temperatures, the oxygen permeability of some of these materials can exceed that of porous membranes. For instance, LiNbO3 (Prod. No. 254290), SrCeO3, and SrTiO3 (Prod. No. 517011) are examples of perovskite-type oxides that have been successfully used for oxygen transport. Membranes created from these materials are dense and as a result, their separation selectivity for oxygen is highly promising. In particular, the oxygen-transport mechanism for these materials involves oxidation and reduction of O2 at the membrane surface, with diffusion of oxide ions and electrons through the dense ceramic matrix, driven by an oxygen potential difference across the membrane. The stability and high oxygen flux potential at high temperatures of these materials, not surprisingly, have provided for their consideration in a number of high-temperature membrane reactor applications, including in oxidation reactions.12,13
As mentioned, ferroelectric perovskite oxides, including BaTiO3 and SrTiO3, exhibit large non-linear optical coefficients, large dielectric constants, and low loss characteristics. Their novel physical properties often result from strong electron–electron interactions. Because these effects are dependent on structure and finite size (e.g., the ferroelectric transition temperature of isolated grains may decrease for average grain parameters of about 200 nm),14,15 considerable effort has been expended in the rational synthesis (in terms of size, shape, and morphology) of pure crystals and of thin films of these ferroelectric oxides.16-18 As compared with bulk ferroelectrics, low-dimensional nanoscale ferroelectric structures may increase the storage density of nonvolatile ferroelectric random access memories (NVFRAMs) by as much as a factor of 5.19
Applications of Perovskite-phase Metal Oxide Materials
The properties and applications20 of a number of important perovskitephase mixed-metal oxides are summarized in Table 1. The uses for these materials are based upon their intrinsic dielectric, ferroelectric, piezoelectric, and pyroelectric properties of relevance in corresponding electronics applications such as electromechanical devices, transducers, capacitors, actuators, high-k dielectrics, dynamic random access memory, field effect transistors, and logic circuitry.1,21,22 All ferroelectric materials are both pyroelectric and piezoelectric with the potential for additional utility based upon these properties.
Materials | Properties | Applications |
---|---|---|
BaTiO3 | dielectric | capacitor, sensor |
(Ba,Sr)TiO3 | pyroelectric | pyrodetector |
PbTiO3 | pyroelectric piezoelectric | pyrodetector, acoustic transducer |
Pb(Zr,Ti)O3 | dielectric pyroelectric piezoelectric electro-optic | nonvolatile memory, pyrodetector surface acoustic wave device, substrate waveguide device |
(Pb,La)(Zr,Ti)O3 | pyroelectric electro-optic | pyrodetector waveguide device, optical memory display |
LiNbO3 | piezoelectric | pyrodetector, surface acoustic wave device |
(LiNbO3/Ti) | electro-optic | waveguide device, second harmonic generation, optical modulator |
K(Ta,Nb)O3 | pyroelectric electro-optic | pyrodetector waveguide device, frequency doubler |
Pb(Mg1/3Nb2/3)O3 | dielectric | memory, capacitor |
For instance, ferroelectric materials can have their polarization reversed (switched) for memory applications at or near TC. In particular, the drive for size reduction in electronic components has led to the development of ceramic capacitors, especially the multilayer ceramic capacitor (MLCC), using ceramic dielectric materials with the highest permittivity values. More complicated multilayer configurations have also been devised to fulfill the growing technological need for increased capacitance within an ever smaller confinement space.23 In recent years, MLCCs possessing a capacitance of 1 to 100 μF have been produced with internal Ni electrodes, which are composed of 500 or more laminated thin dielectric layers of ~2 μm.8
Another area attracting a good deal of interest has been the use of ferroelectric thin films as nonvolatile computer memories.20 Current static and dynamic memory chips lose data they contain when the power to the memory component is interrupted, often resulting in an irretrievable loss of information. Magnetic storage facilities provide one answer to this problem, but the need for fast, lightweight memories with low power consumption integrated into silicon chips is important for some applications. These materials would also in theory be unaffected by radiative and magnetic fields, which can destroy the data stored on a standard memory chip. There are two key designs for a nonvolatile memory which utilize ferroelectric materials, namely lateral configuration and vertical configuration. One of the main features of these architectures is the nondestructive readout method, which has been a general problem for these types of memory devices. In other words, to read the memory cell, it must be poled, which can invariably erase the information the cell contained. This drawback can be overcome by designing a system, so as to subsequently rewrite the information back into the memory once the cell has been initially read.
Another application currently under investigation is the use of ferroelectric materials as electro-optic switching devices for optical computers.13,20 This idea is based upon the ability of a ferroelectric material to change its refractive index under an applied field. The response of this material for electro-optic applications is influenced by many factors such as film thickness, wavelength of the light, and the properties of the electrodes. Another application for thin films would be as read/write optical storage devices. In this case, the material, such as lead lanthanum zirconate titanate (PLZT), is switched between its ferroelectric state and its antiferroelectric state upon the application of a light beam, while the film is simultaneously under the influence of an applied field. The signal-to-noise ratio, which is typically poor for thin films, can be significantly increased in these particular thin PLZT film systems (~0.5 μm) under conditions that optimize interference effects. This type of optical storage device is relatively unaffected by the presence of magnetic fields and thus, can be considered as the optical equivalent of magnetic hard disks in computers.
Controlling the Physical Properties of Perovskite-phase Materials
The physical properties of these materials are often tailored through formation of either nonintegral stoichiometric phases or solid solutions. Because metallic conductivity in perovskites is due to the strong cation-anion-cation interaction, altering the structure of these materials through chemical substitution can have a dramatic effect on local order and the properties of the resulting material. The CaTiO3-SrTiO3 system is a model example.24,25 Strontium titanate is a cubic perovskite whereas calcium titanate (Prod. No. 633801) is an orthorhombically distorted perovskite. In the combined CaxSr1-xTiO3, where Ca+2 is substituted at the Sr+2 site, the value of ‘x’ has a dramatic effect not only on the dielectric constant but also on the phases of the material, which may range from orthorhombic to tetragonal to cubic.26-28 Whether this material undergoes ferroelectric or antiferroelectric phase transitions depends on its exact chemical composition.29 As an example of the significance of this synthetic capability, the availability of nanosized Ca0.7Sr0.3TiO3 particles may enhance the material’s existing usage as an efficient dielectric barrier for the plasma-induced, catalyst-free decomposition of CO2.28
Molten Salt Synthesis of Perovskite-phase Materials
While bulk materials have long been prepared using the molten salt synthesis (MSS) method, the preparation of uniform nanostructures using this technique has only arisen within the current century. Antonietti et al.30 first described a ‘convenient route for the synthesis of nanoparticulate salts, metals and metal oxides’ using concentrated salt solutions and block copolymer surfactants. Research groups in Asia31-33 subsequently demonstrated the high-yield preparation of smooth single-crystalline Mn3O4 (Prod. No. 377473), SnO2 (Prod. No. 549657), and CuO (Prod. No. 544868) one-dimensional nanostructures through the MSS method using NaCl in the presence of a surfactant (such as TWEEN® 80 and Triton® X-100), and found that an Ostwald ripening mechanism (namely the selective dissolution of fine particles and redeposition onto larger particles) was primarily responsible for the nanowire growth observed.
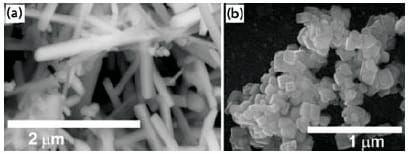
Figure 1.SEM images of BaTiO3 nanorods and SrTiO3 nanocubes.
In our laboratory, the MSS reaction has been used to prepare pristine BaTiO3 nanostructures (including nanowires) with diameters ranging from 50 to 80 nm and with aspect ratios ranging from 1:1 to larger than 25:1, as well as single-crystalline SrTiO3 nanocubes with a mean edge length of 80 nm (Figure 1). The MSS method is one of the simplest, most versatile, and cost-effective approaches available for obtaining crystalline, chemically-purified, single-phase powders in high yield, often at lower temperatures and in overall shorter reaction times with little residual impurities as compared with conventional solid-state reactions.34 The appeal of this technique arises from its intrinsic scalability, generalizability, and facility as well as its fundamental use of salt as the reaction medium.34 In fact, as summarized in Table 2, (i) the identity as well as the size of the anion associated with the salt, (ii) the solubilities/dissolution rates of the constituent components within the molten salt itself, (iii) the precise melting point of either the salt or complex salt mixture used, (iv) heating temperature and duration, as well as (v) the unique morphological (e.g., shape) and chemical composition of the precursors involved are all important, readily controllable factors that can influence the growth rate as well as the resultant structural characteristics (i.e., size, shape, and crystallinity) of the as-prepared particles.34-36

Table 2.Flow chart and factors influencing the MSS method for perovskite-phase material synthesis.
MSS is a simple, readily scaleable (in terms of grams) solid-state reaction in the presence of NaCl and a non-ionic surfactant, i.e., NP-9 (nonylphenyl ether). The following equations summarize a postulated mechanism for the synthesis of these nanomaterials. Here, the term ‘M’ (the metal species) can represent either Ba or Sr, as relevant examples.
MC2O4 → MO + CO + CO2 (1)
MO + TiO2 → MTiO3 (2)
The importance of the experimental conditions used to form these nanostructures cannot be underestimated. It is noteworthy that for BaTiO3 and SrTiO3 in particular, the use of different precursors, such as oxides and chlorides, as opposed to oxalates, produces neither phase-pure titanates nor even nanostructures. Even a slight change of conditions, such as running the reaction at a lower temperature, i.e., 810 °C (below the melting point of NaCl), results in a lack of phase-pure titanates. The thermal effect can be rationalized by the change in the viscosity in the growth medium, which decreases when the temperature increases.
Additional experimental results37 demonstrate that, while maintaining identical experimental conditions (i.e., same ionic strength, temperature, and/or surfactant) but altering the metal precursor used, different shapes of perovskite nanostructures can be synthesized. These data are suggestive of the importance of interfacial energies between the constituents and the salt medium itself in determining the ultimate product morphology obtained. In fact, the shape of a nanocrystal is determined by the relative specific surface energies associated with the facets of the crystal. That is, with barium, nanowires can be fabricated whereas with strontium, nanocubes are normally synthesized. The ultimate shape of a single crystalline nanostructure reflects the intrinsic symmetry of the corresponding lattice.
The MSS method has also been used to prepare a series of single-crystalline Ca1–xSrxTiO3 (0 ≤ x ≤ 1) nanoparticles.38,39 Shapes of the generated Ca1–xSrxTiO3 nanoparticles alter from cubes to quasi-spheres with decreasing x values. Typical nanoparticles (Figure 2) have sizes ranging between 70 and 110 nm, irrespective of the Sr or Ca content. The precise chemistry of the resulting nanoparticles is readily tunable by adjusting the ratio of the reactants. This capability to generate materials of a desired doping level should enable future investigations of the composition-dependent properties of these materials. For instance, nanocubes of SrTiO3, measuring 80 ± 10 nm, show strong first-order Raman scattering, unlike the bulk. Moreover, rapid polarization fluctuations within the nanoscopic ferroelectric regions in these materials can interfere with a polar phonon, resulting in a Fano-like asymmetric line shape in these SrTiO3 nanocubes as well as in Ca0.3Sr0.7TiO3.39
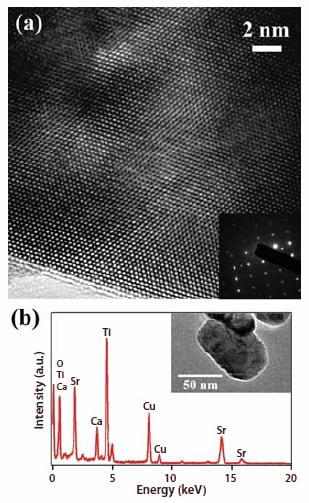
Figure 2.(a) HRTEM image of a Ca0.3Sr0.7TiO3 nanoparticle. Inset of (a) is a SAED pattern of a Ca0.3Sr0.7TiO3 nanoparticle. (b) EDS data of the as-synthesized Ca0.3Sr0.7TiO3 nanoparticle. Inset of (b) is a typical TEM image of the as-prepared Ca0.3Sr0.7TiO3 nanoparticle sample.
Rational control over the actual shape of perovskite metal oxides has recently been achieved for single-crystalline BaZrO3 (Prod. No. 631884) particles40 through the MSS methodology using a NaOH/KOH salt mixture (Figure 3). The evolution of particle morphology from predominantly cubes to a mixture of cubes and spheres and finally to solely spheres has been demonstrated by increasing annealing/reaction times at suitable annealing temperatures, all other parameters being equal. Manipulating the shape of perovskite oxides is of great importance due to their strongly structure-dependent physical properties.41,42 For instance, as-prepared, submicron-scale samples possessed a noticeably higher photoluminescence (PL) efficiency as compared with bulk, and in particular, spheres evinced a better PL signal as compared with cubes.
![BaZrO3 cubes: (a) Typical TEM image; (b) HRTEM image of a portion of the cube in (a) with zone axis corresponding to [001]; (c) SAED pattern of cube sample; (d) EDS. The Cu and C peaks originate from the TEM grid. BaZrO3 spheres: (e) Typical TEM micrograph; (f) HRTEM image of a portion of one of the spheres in (e) corresponding to the zone axis of [011]. Inset of (f) is the associated SAED pattern. BaZrO3 cubes](/deepweb/assets/sigmaaldrich/marketing/global/images/technical-documents/articles/materials-science-and-engineering/photovoltaics-and-solar-cells/fig3.gif)
Figure 3.BaZrO3 cubes: (a) Typical TEM image; (b) HRTEM image of a portion of the cube in (a) with zone axis corresponding to [001]; (c) SAED pattern of cube sample; (d) EDS. The Cu and C peaks originate from the TEM grid. BaZrO3 spheres: (e) Typical TEM micrograph; (f) HRTEM image of a portion of one of the spheres in (e) corresponding to the zone axis of [011]. Inset of (f) is the associated SAED pattern.
In additional experiments,43 we noted that the production of relatively high quality barium zirconate samples was generally favored by high annealing temperatures, slow cooling rates, and overall long reaction times. In terms of optimal overall reaction conditions, the most uniform, crystalline, well-dispersed, and chemically homogeneous BaZrO3 submicron- sized particles were obtained using BaC2O4 (Prod. No. 456004) and ZrO2 as precursors; NaOH/KOH as the molten reaction medium; a molar ratio of BaC2O4:ZrO2:salt corresponding to 1:1:20; a heating rate of 5 °C/min; as well as a reaction temperature of 720 °C. Shorter annealing times (e.g., 30 min) coupled with higher cooling rates (e.g., 100 °C/min) led to the production of smaller-sized cubic particles. By contrast, longer annealing times (e.g., 60-210 min) and/or slower cooling rates (e.g., 5 °C/min) induced particle conversion from cubes to spheres and usually resulted in a mixture of cubic and spherical morphological motifs. Moreover, either increasing the annealing time or slowing the cooling rates resulted in the formation of larger-sized spherical particles.
Future work in this area will involve the detailed investigation of ferroelectricity, piezoelectricity, and paraelectricity at the nanoscale, as well as mechanistic studies of the formation and growth of these nanostructures for the purposes of synthetic optimization. More specifically, these efforts will concentrate on understanding structureproperty correlations in perovskite nanostructures.
References
Para seguir leyendo, inicie sesión o cree una cuenta.
¿No tiene una cuenta?