Poly(Amino Acid) Block Copolymers for Drug Delivery and other Biomedical Applications
Introduction to Polyamino Acids: The Next Best Thing to Proteins
Humankind has utilized protein materials throughout its existence, starting with the use of materials such as wool and silk for warmth and protection from the elements and continuing with the use of recombinant DNA techniques to synthesize proteins with unique and useful properties. Proteins consist of amino acids linked in a predetermined and genetically-prescribed sequence. There are twenty amino acids found in naturally occurring proteins. Commonly known as the standard proteinogenic amino acids, the sequence of these building blocks form the basis of protein function. The specific function of the protein impacts the sequence and abundance of particular amino acids. For example, the protection of the Bombyx mori pupa within the silk cocoon is a primary natural function of silk protein. As can be expected, the very hydrophobic and chemically non-reactive glycine and alanine comprise 70% of the amino acids in silk protein. Enzymes, however, are proteins that perform significantly different functions and, as such, are comprised of a different set of amino acids. Enzymes fulfill biochemical catalytic functions and their amino acid sequence enables catalytic activity through the composition and molecular architecture of the catalytic pocket. The stability of the enzyme is maintained via its unique tertiary structure and the solubility of the enzyme in its (aqueous)medium of activity is guaranteed by functionalized amino acids that form the outside of the three dimensional structure.
This capability to enable unique and highly-targeted biological function makes proteins ideal candidates for drug delivery systems because proteins have the ability to:
- Form an ideal “cradle” for a variety of therapeutic drugs, including delicate hydrophilic proteins or hydrophobic small molecules.
- Interact with, fold, and transport DNA.
- Deliver a therapeutic to a targeted location using site-specific interactions, exploit transmembrane transport mechanisms, and deliver a therapeutic payload to a specific site of action, free of side-effects.
However, our ability to engineer protein structure as well as understanding the infinite resulting interaction possibilities is still in its infancy. Despite of our current understanding of the intricacies of proteomics, the synthesis of fault-free (i.e., sequence-correct) proteins in appreciable amounts is still unresolved.
The limitations in our ability to synthesize proteins tailored for delivery of specific drugs has led researchers to concentrate on “the next best thing”: poly(amino acid)s (PAAs). While this category of polymers is also sometimes referred to as “polypeptides”, this author suggests the use of the term is slightly misleading because “peptide” refers to a polymeric structure with a specific amino acid sequence. However, in the macromolecules considered here, typically only two or three different amino acids are used for synthesis and no specific amino acid sequence is achieved.
PAAs are similar to proteins (and peptides) in that they consist of amino acid building blocks linked by means of amide bonds. This backbone architecture and the use of naturally occurring l-amino acids for their synthesis ensure their biocompatibility. Subsequently, their potential degradation products are l-amino acids, which will be readily absorbed by the body. Most PAAs consist of no more than two different amino acids that are linked to yield either random or block copolymers.
When l-amino acids are used in the synthesis of PAAs, the polymeric products retain the stereoregularity of the monomers. This results in the creation of isotactic PAA polymers. This is structurally very important as the stereoregularity is responsible for the formation of distinct secondary structures. Typically, helices are expected as secondary structures for isotactic polymers. The resulting PAA copolymers also exhibit the chemical characteristics of the l-amino acid building blocks, such as polarity, electrostatic, and solubility behavior. These physical characteristics are exploited when amphiphilic block copolymers are synthesized. Amphiphilic block copolymers readily undergo self-assembly into gels, micelles, membranes, vesicles, nanoparticles, nanogels, and other three-dimensional structures. The synthesis and utilization of PAA block copolymers is discussed in this article.
Synthesis of Poly(amino acid)s
Poly(amino acid)s and their syntheses were discussed in detail by Kricheldorf.1 PAAs are typically synthesized by the ring-opening polymerization of amino acid N-carboxyanhydrides (NCAs) initiated by an amino-terminated reagent, as shown in Figure 1. Results indicate the polymerization follows a living, ring-opening polymerization. This polymerization technique allows for the synthesis of PAA block copolymers as well as random copolymers, making it highly versatile. If the amino acid NCAs are added successively to the initiator (the addition of the NCA of amino acid A followed by the addition of the NCA of amino acid B), AB block copolymers are formed. If the NCAs of the two amino acids are added to the initiator as a mixture, random copolymers are formed. Additional polymer architectures of varying complexity can be achieved due to the living nature of the polymerization.

Figure 1.Schematic of NCA polymerization leading to the synthesis of PAA block copolymers and PAA random copolymers.
When synthesizing PAAs for drug delivery and biomedical applications, it is crucial to tightly control the molecular weight within a narrow range. This can be accomplished by employing living polymerization techniques to produce block copolymers. To do this, Hadjichristidis et al. employed high vacuum techniques to purify the monomers, solvents, and initiator. The polymerization was then performed in a specialized vessel that guaranteed absolutely anhydrous conditions.2 Deming et al. developed syntheses that use transition metal catalysts to gain control over the molecular weight and polydispersity of the synthesized PAAs.3 Our own studies have shown that the first few addition reactions (i.e., the addition of the first few amino acid building blocks to the initiator) are most crucial to controlling the reaction and producing PAAs of the desired molecular weight and polydispersity.4 In the beginning of the polymerization, the nascent oligomers are not yet long enough to form a helical structure, but tend to form β-sheets due to strong hydrogen bond interactions. The rigidity of these structures suppresses the ability of the growing chain to undergo continued nucleophilic attacks on NCA monomers and these small β-sheet structures have a tendency to precipitate out of the polymerization solution, effectively stopping the polymerization.5 If the β-sheet formation is not kept under control, the resulting final product consists of a few long chains that exceed the intended chain length as determined by the Monomer:Initiator ratio and low molecular weight oligomers.
It was found that urea effectively suppresses the hydrogen bond formation between nascent PAA chains. As a result, the premature precipitation of product is suppressed. This allows for the polymerization to follow a living mechanism.4 Because urea suppresses the formation of β-sheets, there is sufficient time for the amino acid chains to reach a length of about 10 repeat units, enabling formation of α-helices. Once the β-sheet stage is overcome, polymerization proceeds in a controlled manner, where chain ends remain reactive (living) and block copolymers can readily form (Figure 1).
Poly(amino acid) Block Copolymers: Concepts for Drug Delivery Systems
Amino acids have diverse chemical properties and can be subdivided according to the nature of their side chains, including those that are aliphatic, aromatic, sulfur-containing, and those that contain ionizable groups. When designing a PAA for use in drug delivery, amino acids are selected based on chemical properties that meet the needs of both the drug and the solvent environment. Typically, amphiphilic block copolymers are formed from a hydrophilic (ionizable) and a hydrophobic amino acid (Figure 1). This amphiphilicity will induce self-assembly of the block copolymer when exposed to a selective solvent.6 In the case of drug delivery, where polymers are exposed to an aqueous environment, the hydrophilic segment of the block copolymer forms the outer layer or corona of the self-assembled structure, while the hydrophobic block self-assembles into a tightly packed structure that functions as a “cargo compartment” for hydrophobic drugs (Figure 2A).
Deming et al. studied the self-assembly and packing of PAA diblock and triblock copolymers and provided an excellent model to illustrate the polymer interactions and their subsequent self-assembly (Figure 2).7 Specifically, they studied copolymers consisting of poly(l-Leu), a hydrophobic amino acid and poly(l-Lys), an ionizable amino acid. Polymers of the following general structures were prepared: diblock copolymers: p(l-Lys)-block-p(l-Leu) and triblock copolymers: p(l-Lys)-blockp(l-Leu)-block-p(l-Lys), and individual blocks varied in length. The resulting block copolymers have a positively charged p(l-Lys) block that contributes to its solubility in water and where it forms a distorted (stretched) random coil. The hydrophobic p(l-Leu) block forms an α-helix as a secondary structure that is maintained when exposed to an aqueous environment. In the case of the triblock copolymers, there are two p(l-Lys) blocks that are adjacent to the p(l-Leu) block.
When used as drug delivery vehicles, amphiphilic diblock copolymers generally self-assemble into classic spherical polymeric micelles upon exposure to a selective solvent such as water (Figure 2A). In most cases, the hydrophobic segment packs tightly to avoid contact with the water and the hydrophilic segment readily solubilizes to form a shell or corona around the hydrophobic core. However, in the case p(l-Lys)-block-p(l-Leu) diblock copolymers the very stable p(l-Leu) helical structure, assembles into a stiff rod shape, which impedes the formation of a spherical packing structure and a flat lamellar packing of the rods (Figure 2B) predominates. The rods line up in parallel and are held in place through hydrophobic intermolecular interactions. However, the hydrophilic blocks with increasing chain lengths assume a stretched random coil configuration and fill increasing molar volumes. In addition, electrostatic repulsion between the positively charged p(l-Lys) chains renders the situation even more energetically unfavorable. As the chain length of the hydrophilic blocks increases, curved membranes are formed. These are the result of asymmetric packing of the hydrophilic blocks (Figure 2C). The resulting increase in corona volume is energetically favorable. Structures with curvature in two directions can give rise to the formation of polymeric giant unilamellar vesicles. The repulsive effect between the p(l-Lys) blocks can also be resolved by twisting the plane of the p(l-Leu) blocks into fibrils, (Figure 2D). While the helices of the p(l-Leu) blocks maintain parallel packing, the hydrophilic blocks gain volume by expanding around the forming fibrils which extend in one direction only.

Figure 2.Self-assembly arrangement of PAA di- and triblock copolymers into (A) spherical micelles; (B) flat membranes; (C) curved membranes, and (D) fibrils, with the cross section extending out of the plane of the paper and the insert depicting how the helices assemble into twisted fibers. Reprinted with permission from Ref. 7, Copyright 2004, American Chemical Society.
In the case of p(l-Lys)-block-p(l-Leu)-block-p(l-Lys) triblock copolymers, where the two hydrophilic blocks are covalently attached to the hydrophobic core curved structures (Figure 2C) will not form. Instead, asymmetric packing of the p(l-Lys) blocks is impossible and fibrils readily form. Both the curved and the fibrilar structure models can be used to explain the gelation behavior observed for the p(l-Lys)-block-p(l-Leu) and p(l-Lys)-block-p(l-Leu)-block-p(l-Lys) block copolymers.8 Due to their amphiphilic nature, these copolymer systems can be readily loaded with hydrophobic (in the core) as well as hydrophilic (in the corona) drugs.
One can further expand on the above concept of PAA-based drug delivery systems, by using a macroinitiator to start the ring opening polymerization of amino acid NCAs. Poly(ethylene glycol) (PEG), is one of the most thoroughly investigated biocompatible polymers. With its large excluded volume, PEG conveys a “stealth character” to the structures to which it is attached. This essentially makes the structures “invisible” to adjacent protein molecules. The effectiveness of PEG to reduce protein interaction has been explained in physico-chemical terms by its low interfacial free energy in water and by its unique coordination with water molecules, as each PEG repeat unit coordinates with two to three water molecules. PEGylated PAA structures are used as drug delivery systems, employing polymeric micelles or nanoparticles with PEG to form the corona. They are also used to biocompatibilize surfaces of implantable devices intended to be in contact with protein-containing (bodily) fluids. In the case of drug delivery systems, PEGylation prevents uptake by the reticuloendothelial system and PEG-coated surfaces are efficient in suppressing biofouling.
The synthesis of PEGylated PAAs follows the scheme presented in Figure 1, with R representing NH2-terminated PEG. For biomedical applications, PEGs with molecular weights of 2,000 to 10,000 Da are typically used to ensure renal clearance of degradation products. It is also possible to use bifunctional, α,ω-diamino PEGs as macroinitiators. The resulting block copolymers will have the PEG block in the middle, allowing the formation of interesting self-assembled molecular architectures, such as flower-like micelles.

Figure 3.Surface modification with PEGylated PAA. A) The multi-point attachment of the PAA chain to the surface (e.g., by the formation of covalent bonds between l-Cys (yellow) and a gold surface) with the PEG-block (blue) extending away from the surface, thereby conveying a stealth character. B) Attachment of amino acid oligomers by single-point attachment and their subsequent modification with PAA-based polymeric micelles. The X indicates reactive terminal side groups, e.g., amino groups in l-Lys. A covalent bond is established between the PAA oligomer and the PAA polymeric micelle.
PEGylated PAAs can be attached directly to a surface and this surface attachment can be utilized for drug delivery applications.9 The attachment of PEGylated PAAs containing (l-Cys) repeat units can be achieved using gold-covered surfaces.10 To do this, l-Cys NCA is typically copolymerized with another amino acid (shown in green in Figure 3A), such as l-Lys NCA or l-Glu NCA, to achieve the optimal spacing (dilution) of the reactive l-Cys residues. The l-Cys repeat units react instantaneously with gold surfaces. The repeating l-Cys units in each block copolymer chain result in multiple anchor points to the surface (Figure 3A).
Because the PAA block functions as a multivalent anchor, the PEG block is free to extend away from the surface upon exposure to an aqueous environment. This architecture accomplishes two objectives: 1) PEG is strongly attached to the surface through multiple covalent thiol-gold linkages, and 2) the PAA block remains closely bound to the surface. These two factors contribute to the overall biocompatibility of the surface by masking “holes” that may form between individual PEG chains. It is well known the formation of dense polymer brushes is inherently difficult on surfaces using a “grafting to” method. This is because the concentration gradient built up by the already attached chains provides a strong kinetic hindrance against additional grafting. Furthermore, the large excluded volume that PEG exerts in an aqueous environment, while beneficial for preventing protein interactions, hinders the formation of dense PEG brushes in “grafting to” procedures even further. Figure 4 shows complete coatings are achieved on gold surfaces with both, PEG-block-p(l-Glu)110-block-p(l-Cys)10 and PEG-block-poly((l-Glu)110-co-(l-Cys)10). The polymer architecture, PAA random copolymer vs. block copolymer, has little to no influence on the polymer morphology on the surface. The coatings are homogenous and pinhole-free.
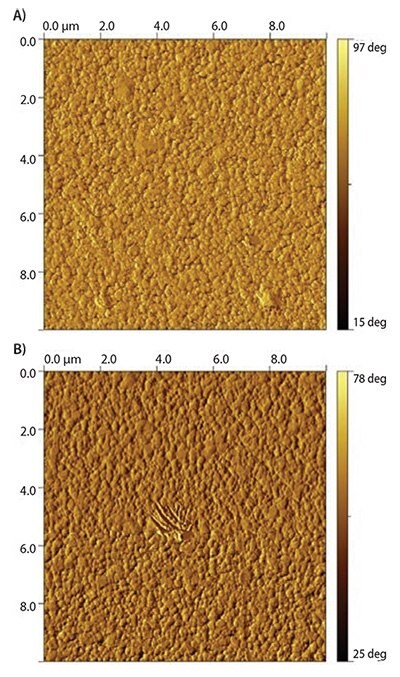
Figure 4.10×10 μM AFM phase images of gold surfaces coated with PEG-b-p(l-Glu)110-blockp(L-Cys)10 (A) and PEG-block-p((l-Glu)110-co-(l-Cys)10) (B).
Another concept combines surface coating with the option of decorating the surface with PAA-based polymeric micelles that could be drug-loaded; the concept is schematically depicted in Figure 3B. In this procedure, oligo-(l-Lys) was attached covalently to the gold surface by a terminal thiol group, which is the result of using cysteamine as the initiator in the ring-opening polymerization of l-Lys NCA. Subsequently, the surface was decorated with micelles prepared from PEGylated PAAs (HOOC-CH2-PEG114-block-poly(Bz-l-Glu)13) that carry carboxyl groups in their corona and were, therefore, reactive toward p(l-Lys).11 Terminal carboxyl groups in the poly(Bz-l-Glu) side chains are protected as a benzyl ester to achieve the amphiphilicity that drives micellization. The resulting micelles are maintained due to the strong hydrophobic interaction of the benzyl groups in the micelle core. The micelles were covalently attached to the poly(l-Lys) using an amide linkage that formed between the terminal carboxyl groups of the polymeric micelles and the amino functions of poly(l-Lys), shown in Figure 5.

Figure 5.10×10 μM AFM phase image of a gold surface coated with thiol-terminated p(l-Lys)15 and decorated with polymeric micelles formed by HOOC-CH2-PEG114-block-p(Bz-l-Glu)13.
Summary
The synthesis and application of PAA block copolymers in drug delivery and surface modification of biomedical products was discussed. Various self-assembly structures, including spherical polymeric micelles and membranes which enable gel formation, were examined. It was shown amino-terminated PEG can be used as a macroinitiator in the synthesis of PAAs, yielding block copolymers. PEGylated PAAs also self-assemble into polymeric micelles. Furthermore, if the PAA segment contains an amino acid that is reactive toward a surface (e.g., l-Cys reacts instantaneously with gold), the PAA block can be employed as a multi-point anchor to attach PEG covalently in a dense packing by a “grafting to” method to a surface. In a different surface modification approach, PAA oligomers attached covalently to a surface can be further decorated with PAA polymeric micelles, enabling attachment of a drug delivery system to a surface.
References
To continue reading please sign in or create an account.
Don't Have An Account?