Recent Advances in 3D-Bioprinting-based Drug Screening: A Mini Review and Perspective
Xiuli Zhang2,*, Yong Luo1, Long He1
1State Key Laboratory of Fine Chemicals, Department of Pharmaceutical Sciences, School of Chemical Engineering, Dalian University of Technology, 116024, Dalian, Liaoning Province, China
2Jiangsu Key Laboratory of Neuropsychiatric Disease and College of Pharmaceutical Science, Soochow University, 215127, Suzhou, Jiangsu Province, China
Introduction
Three-dimensional culture1 is becoming an increasingly accepted paradigm for cell cultivation due to its ability to simulate the in vivo microenvironment of cells in three dimensions. This fosters better cell viability, particularly for primary cells, and is easy to standardize.2 It is currently a significant focus for the FDA. Three-dimensional (3D) culture can be divided into suspended 3D culture3 and scaffold-based 3D culture.4 The latter is a hot research topic, focusing on developing advanced biomaterials. By embedding cells within such materials, phenotypes can be more apparent, and the cells’ functions can be more robust.5
Biomaterials can be designed to be printable.6 3D bioprinting technologies can customize the shape and internal structure of such materials, as well as precisely 3D position the cells in the materials, thereby achieving highly controllable three-dimensional cell co-culture, which has the potential to simulate tissues and organs.7 Using 3D bioprinting technology to construct three-dimensional cell co-culture systems is an advanced model for drug screening due to its highly biomimetic nature. This review paper highlights recent progress in 3D bioprinted tissue models and summarizes the advancements of 3D bioprinting in drug discovery. Finally, this paper discusses the future of 3D bioprinting-based drug discovery and how it compares to other biomimetic techniques like organoids8 and organ chips.9
3D Bioprinted Tissue Models
3D-bioprinting-based drug screening relies on 3D bioprinted tissue models that are rapidly advancing, such as 3D-bioprinted liver. The liver’s intricate vascular network is crucial for substance exchange during drug metabolism. A good goal is the development of a vascularized in vitro liver model using 3D bioprinting. In a recent study, Kang et al. achieved this by creating a heterogeneous, multicellular, multi-material liver lobule array.10 The team designed a pre-defined 1 mm structure, encompassing a 150 μm diameter central vein channel, high-density liver cells with embedded endothelial cells, 10 μm resolution micro-nozzles, and an external endothelial lining to form a luminal tube. Using a battery of tests, the researchers evaluated the formation status, structural integrity, mechanical performance, liver function expression, and amiodarone-induced liver toxicity. The bioprinted body yielded ideal results in all tests, indicating its potential as an in vitro liver construction model.
In recent years, 3D coaxial printing technology has shown great potential in directly manufacturing injectable hollow structures. In particular, Singh11 and others have made significant progress by developing a decellularized kidney-derived bioink, that is combined with a mixture of sodium alginate, renal proximal tubule epithelial cells (RPTECs), and human umbilical vein endothelial cells (HUVECs) for bioprinting kidney tubular structures. Using a coaxial nozzle, their bioprinter can simultaneously extrude bioink containing free substances and cells. After crosslinking the shell, the middle part is removed, forming complete single or double-layer tubular structures. The RPTEC and HUVEC tubes have also been successfully transplanted into the peritoneal region of immunodeficient mice and implanted into host kidney tissue. Bioprinting of multi-tubular tissue structures is a promising strategy; most importantly, it is applied in drug reabsorption and excretion and the replacement of damaged kidneys. Deng et al. introduced a novel strategy for constructing hollow tubular structures. By employing a time-dependent crosslinking approach, they successfully controlled the thickness of the tubular wall. An important advantage of this method is its compatibility with cell seeding, allowing for the replication of various tubular structures relevant in vivo, such as blood vessels, renal tubules, etc.29
Faheem Ullah et al. describe the successful synthesis of a novel bioink, PEO-CS-PMMA, used to construct skin tissue structures through 3D bioprinting technology.12 The bioink was obtained by copolymerization of PEO, chitosan, and PMMA to improve its density, viscosity, and printing ability while maintaining thermal stability. The resulting bioink was highly organized and porous, allowing it to absorb water and release growth factors and bioactive substances to promote healing and recovery.
Ma et al. described a method to cross-link bioinks with Ca2+ to obtain gelatin-alginate for a 3D bioprinted hydrogel scaffold with good stability and biocompatibility.13 The scaffold can mimic the physical microenvironment of dermis and subcutaneous tissue by encapsulating adipose stem cells to promote their proliferation and migration. The unique gradient composite scaffold promotes angiogenic and wound healing effects by enhancing the paracrine secretion of adipose stem cells. This 3D-printed scaffold provides a continuous and unique structure for adipose stem cells to promote skin regeneration and can be expanded to complex tissue injury models.
Lee and colleagues utilized fiber-optic-assisted bioprinting to cross-link methacrylate-based hydrogels (GelMA) and construct biofunctional cell-loaded structures by selecting appropriate photocrosslinking process conditions, such as printing temperature.14 Their experiment induced C2C12 mouse myoblasts and human adipose stem cells (HASCs) to construct cell-loaded structures for muscle regeneration. Compared to traditional printing processes, the cell-loaded structures showed a higher degree of cellular alignment and myogenic activity. Models constructed using HASC also demonstrated superior muscle regeneration compared to those constructed without topographical cues. However, cellular activity factors like cellular metabolism remain challenging, leading to insufficient oxygen transport in the 3D cellular constructs for muscle tissue regeneration. Hwangbo and colleagues addressed this issue by introducing photosynthetic cyanobacteria (Alcaligenes longum) into Gelma bioink. They used in situ electronic bioprinting to prepare cell-loaded scaffolds that promote cellular arrangement and muscle formation.15 They concluded that this approach could be an effective treatment for severe skeletal muscle defects by evaluating the combined effects of bioactive components in bioinks and bioprinting that simultaneously support electronic fields.
Bionic spinal scaffolds, based on 3D bioprinting technology, can effectively mimic the shape and structure of spinal cord tissue, and promote the healing of spinal cord injuries. However, they have yet to be successful in mimicking the biological functions of the spinal cord due to insufficient electrical conductivity. Gao et al. developed a new conductive hydrogel, PEDOT:LS, utilized in a bionic scaffold based on GelMA, hyaluronic acid methacrylate (HAMA), and poly (3,4-ethylene dioxythiophene) to mimic the spinal cord’s electrical conductivity.16 The conductive hydrogels showed similar mechanical properties to natural spinal cord tissue, and neural stem cell (NSC) culture showed high survival rates. Compared to non-conductive scaffolds, the 3D bioprinting-based conductive scaffolds significantly promoted neuronal differentiation of NSCs in vitro and improved hind limb motor function recovery in a rat spinal cord complete transection model. Zhang et al. introduced inorganic calcium silicate (CS) nanowires in bioink for innervated bone regeneration through 3D bioprinting technology.17 They established a model that combines nerve and bone-associated cells printed in an orderly fashion, encapsulated by CS nanowires that enhance long-term viability and proliferation of encapsulated cells and promote osteogenesis and neural differentiation. By introducing inorganic nanomaterials into bioinks and 3D bioprinting technology, this work provides a potential strategy for complex bone tissue regeneration.
The combination of 3D bioprinting technology, fused deposition modeling (FDM) technology, and light-cured additive manufacturing (DIW) has significantly advanced cartilage and bone tissue engineering. Chen et al.18 prepared a biphasic bone scaffold using alginate-gelatin hydrogel (A-G) as the bioink and poly-caprolactone (PCL) to improve mechanical stability and overall performance. The bone phase of the scaffold was enhanced by integrating hydroxyapatite into the PCL, resulting in improved bioactivity. Notably, the physical and biological evaluation of the scaffold in both the bone and cartilage phases confirmed its sound biological effects in both the short and long term, making it a promising interface material between cartilage and bone.
3D Bioprinting for Drug Screening
Compared to 3D-bioprinting-based tissue models, 3D-bioprinting-based drug screening is still in its infancy. Hong et al.19 used extrusion-based 3D bioprinting technology to fabricate a gelatin-sodium alginate-based construct embedding MCF-7 cells and observed the auto-aggregation of MCF-7 cells into spheroids. These spheroids were found to be able to maintain their drug-resistant phenotype of CD44 high /CD24 low /ALDH1 high and exhibited higher expression levels of drug resistance markers, such as the GRP78 chaperon and ABCG2 transporter. This superior resistance was proven through camptothecin and paclitaxel.
Li et al. developed a 3D-printed breast cancer model with hydroxyethyl cellulose/alginate/gelatin (HCSG) composite biomaterial.20 They first demonstrated the potential of 3D bioprinting technology used in a structure–activity relationship study, by investigating the pharmacodynamics of 13 amino acid-based flavone phosphoramidates. Compared to 2D monolayer models, 3D printed models presented different pharmacological activity characteristics (Figure 1).

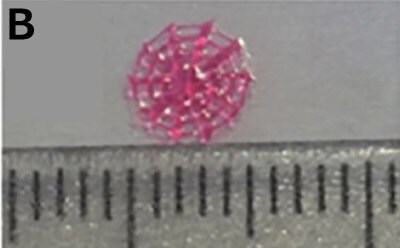
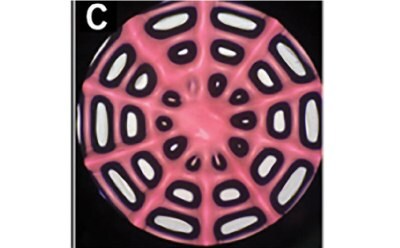

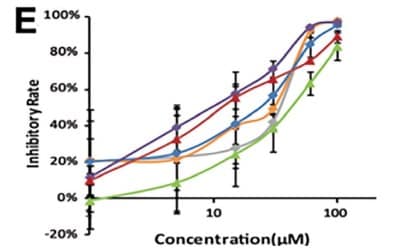
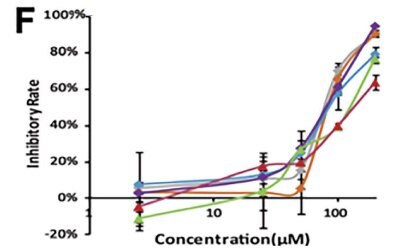
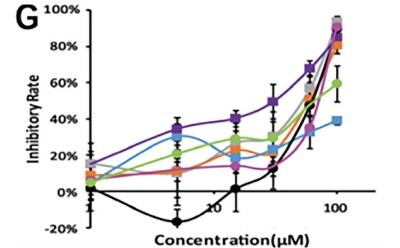
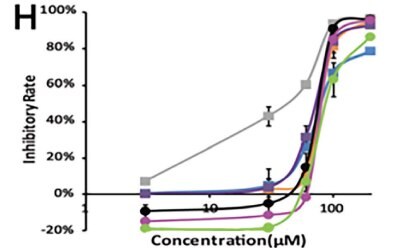
Figure 1. 3D printed breast cancer model. A) Workflow diagram. B-C) Schematic diagram of 3D printed“Spider web” HCSG breast cancer model. D) Investigating pharmacodynamics of 13 amino acid-based flavone phosphoramidates with 2D monolayer model and 3D printed model. Copyright © 2020, Zhejiang University Press.
Qiong Liu et al.21 used sacrificial extrusion-based 3D bioprinting technology to create tubular structures inside the GelMA gel and let cholangiocarcinoma (CCA) cells attach to the surface of the tubular structure to simulate human biliary tract cancer. In this 3D printing model, the authors observed that CCA was overgrowing in a thickening manner, generating bile duct stenosis, which was expected to be analogous to the in vivo configuration. Further, CCA cells showed higher sensitivity to anti-tumor drugs than conventional 2D cell models, providing a novel drug screening model for treating bile duct cancer. Decellularized extracellular matrix (DECM) is often added to printable biomaterials to increase biocompatibility.22
Janani et al.23 developed two different bioinks based on tissue specific DECM of liver cells. These bioinks had excellent printability and rheological properties and could support the printing of liver lobules’ parenchymal and non-parenchymal cells. The print model showed dose-dependent clinical liver toxicity reactions to acetaminophen and troglitazone, providing a powerful platform for liver toxicity screening. Human-induced pluripotent stem cells (hiPSCs) are a source of cells that can be differentiated into normal human cells.24 The 3D bioprinting technology can replicate human tissues or organs if normal human cells are used. He Jianyu et al.25 printed hiPSC-induced-hepatocytes to construct a human liver model. Compared to traditional 2D culture models, hiPSC-induced hepatocytes in this model showed better mRNA expression related to human liver-specific functions and exhibited liver toxicity induced by acetaminophen.
Rency Geevarghese et al. developed a bioink containing several components, including alginate, diethylaminoethylcellulose, gelatin, and collagen peptides, and performed experimental analyses to ensure its suitability for constructing 3D models.26 The team evaluated the effectiveness of their models by examining the growth and proliferation of A549 cells encapsulated within 3D bioprinted structures. They demonstrated that 3D bioprinting-based tissue models are a more effective means of drug screening, providing a platform that mimics the characteristics of native tissues and offers a superior alternative to current 2D cell toxicity testing. The researchers qualitatively and quantitatively validated the potential of their 3D bioprinted structures as drug screening models using apoptosis assays and MTT assays. In conclusion, their findings suggest that 3D bioprinting has the potential to revolutionize drug screening practices, providing a more effective and reliable means for evaluating drug efficacy.
Zicheng Fan et al. utilized dot extrusion printing (DEP) technology to produce precise positioning and adjustable size of hepatocyte-laden gelatin methacryloyl (GelMA) hydrogel microbeads and HUVEC cell-containing gelatin microbeads, creating endothelialized liver lobule-like structures.27 Through experimental results, the team found that the appropriate GelMA concentration mimicked the naturally occurring microenvironment and enhanced the growth and propagation of hepatocytes while providing a suitable surface for tight junctions and the growth and proliferation of endothelial cells. Compared to two-dimensional hepatocellular carcinoma cell models, the model obtained through DEP technology also enhanced the efficacy of the antitumor drug sorafenib. The HUVEC-formed endothelial barrier prevented the spread of sorafenib. The authors concluded that DEP technology could potentially construct 3D models of stromal and cancer cells to promote their growth and multiplication, creating a complex tumor microenvironment. The endothelialized hepatocellular carcinoma models based on 3D bioprinted liver lobule-like structures can simulate pharmacodynamics similar to real-life conditions, making them a valuable tool for drug testing.
Xue Liu et al. developed a reproducible method for inducing endothelial cells of different complexity through 3D bioprinting of human keratinocytes, fibroblasts, pericytes, and multifunctional stem cells to fabricate skin models of varying physiological complexity.28 These included human epidermis, non-vascularized, and vascularized models utilized for high-throughput drug screening on multiwell plates. This innovative combination of 3D cell culture and physiologically relevant 3D tissue models enabled high-throughput pharmacological studies for the first time. The researchers also evaluated the model for the efficacy of potential drugs for AD, with their results correlating with clinical treatment data, highlighting the model’s importance in 3D tissue disease modeling and drug screening. Their research offers a valuable tool for future pharmacological studies on multiwell plates and further contributes to 3D tissue disease modeling.
Perspectives
Besides 3D bioprinting, organ-on-a-chip and organoid are two other biomimetic techniques that can be used in drug discovery.
Organ-on-a-chip technology comprises micro-fabricated cell culture systems that accurately mimic the structure, function, and microenvironment of human organs. These microdevices are composed of chambers, microfluidic channels, and sensors that can simulate the complex physiological environment of human organs such as the heart, liver, lung, kidney, and brain. Essentially, the chip mimics the circulation of the blood, the metabolic activities of the cells, and the mechanical forces that act on the organ. Organ-on-a-chip technology has several advantages over traditional cell culture and animal models.
Firstly, it can recreate the complex microenvironments of the organs, including the physical and chemical cues that regulate the growth and function of cells. Secondly, it allows for real-time monitoring and analysis of cellular and molecular changes, enabling researchers to directly observe drug responses and disease progression. Thirdly, it provides a more cost-effective and ethical alternative to animal testing, with the possibility of personalized medicine through patient-derived cells.
Organoids are three-dimensional structures that closely resemble the gross and microscopic anatomy of an organ or tissue. They are formed from self-organizing stem cells that differentiate to form various cell types found in the desired organ, giving rise to complex functional tissues. Organoids can be created from different types of stem cells, including embryonic stem cells, induced pluripotent stem cells, and adult stem cells. They are valuable tools in drug development, disease modeling, regenerative medicine, and personalized medicine. Organoids have significant advantages over traditional two-dimensional cell cultures and animal models in studying human disease and testing drugs. They provide an accurate representation of the actual organ, including the microenvironment and tissue architecture, allowing for more accurate and reliable drug screening.
Moreover, they minimize the need for animal testing and provide a powerful tool for elucidating the mechanisms of disease progression and novel therapies.
Organoids have been successfully generated from various organs, such as the brain, liver, kidney, pancreas, and intestine, among others. These organoids are being progressively improved by incorporating multiple cell types, establishing vascularization, and introducing functional readouts to approximate organ physiology more closely. With continued advances in stem cell technology and organ engineering techniques, organoids are expected to revolutionize biomedical research and contribute to the development of precision medicine.
3D-bioprinted organs have massive potential in organ transplantation and are perfect alternative models to animal experiments. However, generally, the 3D bioprinted organs are larger in size as compared to organs-on-a-chip and organoids, which limited their use for large-scale screening. The increasing resolution of 3D bioprinting will alleviate this situation. Further, in the future, the 3D bioprinted mini-organ can also be combined with organ-on-a-chip and organoids to develop more biomimetic models for drug discovery.
Acknowledgments
This work was supported by the Jiangsu Key Laboratory of Neuropsychiatric Diseases (BM2013003, ZZ2009), the Hunan Key Laboratory for Bioanalysis of Complex Matrix Samples (2017TP1037), Shenzhen Fundamental Research and Discipline Layout project (No. JCYJ2018050815247476), and the National Key Research and Development Program of China (No. 2017YFC1702001).
REFERENCES
Para seguir leyendo, inicie sesión o cree una cuenta.
¿No tiene una cuenta?