Materials for Clean H2 Production from Bioethanol Reforming
Hong Xie, Eric Weitz, Kenneth R. Poeppelmeie
Center for Catalysis and Surface Science, Department of Chemistry Northwestern University
Introduction
Hydrogen is one of the most important resources in providing food, fuel, and chemical products for our everyday life. Sustainable catalytic hydrogen production from bioethanol has gained significant attention in recent years due to globally diminishing fossil fuel supplies, which have necessitated the search for new chemical feedstocks. Ethanol has been considered as one of the most promising renewable energy resources since it has low toxicity and can be safely stored and easily transported. Bioethanol is the ethanol produced by fermentation of biomass materials including sugar cane, corn, and lignocellulose. Catalytic steam reforming (SR) of ethanol has been extensively investigated for hydrogen production in the past decade. These SR reactions are commonly carried out using heterogeneous catalysts, which include an active phase of nanosized metal particles on an oxide support. This article reviews the catalytic process for bioethanol reforming with a focus on supported nanoscale rhodium catalysts.
Thermodynamics for Ethanol Steam Reforming (SR)
The overall SR of ethanol can be simplified as: C2H5OH+ 3H2O → 6H2 + 2CO2. A typical reaction mechanism for ethanol SR reaction is presented in Figure 1. Ethanol conversion by SR may include1-3:
- ethanol dehydrogenation to form acetaldehyde
- acetaldehyde decarbonylation to produce CH4 and CO
- CH4 reforming, i.e., CH4 + H2O → CO + 3H2
- the production of H2 through a water-gas-shift (WGS) reaction: CO + H2O → CO2 + H2.
The goal for the SR process is to obtain a high yield of H2 and a high selectivity toward CO2, since a total of 6 moles of H2 can be obtained from ethanol for every 2 moles of CO2 produced. The CO generated in the SR process is undesired, as it can be poisonous to the catalysts or the electrode in fuel cell applications. Therefore, the demand of high CO2 selectivity makes it necessary to couple SR reaction with WGS reaction.

Figure 1.Reaction mechanism scheme of ethanol steam reforming
Depending on the catalysts and reaction conditions, the actual ethanol SR reaction may undergo different reaction pathways such as ethanol dehydration and decomposition pathways to generate undesired byproducts of CO, CH4, and C2H4, etc. that may lead to coke formation and consequently lower H2 production. The main reactions that may contribute to coke formation are: ethanol dehydration to ethylene followed by polymerization to coke; Boudouard reaction, i.e., carbon monoxide decomposition to form carbon dioxide and coke; and methane decomposition to form coke and hydrogen. In addition, catalyst sintering under SR reaction conditions can also lead to deteriorated catalytic performance by increasing the active phase particle sizes and decreasing the amount of available surface active sites.
Catalysts play a decisive role in the reaction stability, ethanol conversion, and H2 selectivity for ethanol SR reactions. It is, therefore, crucial to develop suitable catalysts that will maximize H2 yield, and inhibit CO production and coke formation that will deactivate catalysts.
Rh-supported Catalysts
Supported transition metal catalysts such as Co and Ni catalysts have been investigated for ethanol reforming reactions.4-6 However, compared to transition metal catalysts, supported noble metal catalysts have shown greatly enhanced performance at much lower metal loadings for the SR of ethanol (Table 1). Aupretre et al.7 investigated the catalytic performance of γ-Al2O3 supported Rh, Pt, Pd, and Ru catalysts with metal loadings of 0.67−1 wt% for the ethanol SR reaction at 700 °C. The 1 wt% Rh catalyst proved to be the most active and selective of these noble metals. Breen et al.8 also reported that Rh/Al2O3 ( Product No. 212857) showed a higher ethanol conversion than Al2O3 ( Product No. 202606) supported Pd, Pt, and Ni catalysts under the same reaction conditions from 400−700 °C. Frusteri et al.1 studied MgO ( Product No. 529699) supported Rh, Pd, Ni, and Co catalysts in the SR of simulated bioethanol at 650 °C. Again, the Rh/MgO showed the best performance in terms of reaction activity and stability. Furthermore, the Rh/MgO catalyst was most resistant to coke formation and metal sintering. In contrast, Ni, Co and Pd catalysts deactivated mainly due to metal sintering. Rioche9 et al. reported the SR of ethanol from 664−779 °C over Pt, Pd and Rh catalysts supported on Al2O3 and CeZrO2 ( Product No. 634174). Only the Rh-based catalysts efficiently converted the ethanol over the temperature range investigated. The Ptand Pd-based catalysts were significantly less active.-The high SR reaction activity of the Rh catalysts is due to the fact that Rh favors C−C bond cleavage compared to other noble metals. Duprez et al.7 proposed a bi-functional mechanism where the hydrocarbon would be activated on the metal phase while the water molecule would be activated on the support as hydroxyl groups. Owing to this effect, oxide supports with enhanced surface oxygen mobility are expected to have improved SR reaction activity.
Support Effects
Rhodium is the most active metal for ethanol SR (Table 1). However, the support also plays a significant role in determining the catalytic performance of the catalysts for ethanol SR reaction (Table 2). The use of CeO2−ZrO2, a redox mixed oxide, led to higher H2 yields as compared to the Al2O3-supported catalysts.9 Roh et al.10 studied Rh catalysts on various supports, including Al2O3, MgAl2O4 ( Product No. 677396), ZrO2 ( Product No. 204994), and ZrO2−CeO2 for ethanol SR reaction. For the 1 wt% Rh catalysts, at temperatures ≤450 °C, the reaction activity was found to decrease in the order: Rh/ZrO2−CeO2 > Rh/Al2O3 > Rh/MgAl2O4 > Rh/ZrO2. The 1% Rh/ZrO2−CeO2 catalyst exhibited the highest CO2 selectivity up to 550 °C. At 450 °C, the CO2 selectivity of 52% was at least 3 times higher than all other tested catalysts. Furthermore, the 1% Rh/ZrO2−CeO2 catalyst exhibited the highest H2 yield among all the catalysts at temperatures below 500 °C.
Generally, a good SR catalyst support should favor Rh stability with minimum Rh sintering. In addition, water should be activated on the support as mobile surface hydroxyl groups to promote reaction with CHxOy fragments adsorbed on Rh species. Finally, it should favor ethanol dehydrogenation to acetaldehyde instead of ethanol dehydration to ethylene in order to prevent coke formation. In fact, the acidic Al2O3 support is very active for the dehydration reaction leading to the formation of ethylene,8 which is undesirable in ethanol SR. Over a CeO2 support, acetaldehyde can be formed via the dehydrogenation of ethanol.3 CeO2 ( Product No. 211575) can promote the redox reaction of rhodium due to its high oxygen storage capacity associated with the fast Ce4+/Ce3+ redox process.10 ZrO2, with its high thermal stability and tunable acidic and basic properties,11 has also been used as a catalyst support for ethanol SR reactions. Zhong et al.12 studied Rh catalysts supported on ZrO2-based oxides; in their study, pure ZrO2 resulted in higher H2 yield compared to the binary metal oxide supports of ZrO2 with Al2O3, La2O3, or Li2O at 300 °C. The addition of ZrO2 to CeO2 can enhance the oxygen storage capacity, the reducibility of Ce4+, and thermal stability of CeO2.13 It is possible that ZrO2−CeO2 may transfer mobile oxygen species to Rh to enhance CH4 reforming and subsequent H2 production, while the oxygen vacancies would be replenished by water molecules from the redox cycle during the SR of ethanol.7,10 Among the investigated supports, CeO2, MgO, and ZrO2 are the most suitable catalyst supports for effective ethanol SR with Rh catalysts.
A. H2 yield (g h-1 g-1 catalyst)
B. Ethanol conversion based on CO + CO2 formation
C. CO2 yield
A. H2 produced/ethanol feed (mol/mol)
B. CO2 yield
C. 99 wt% ZrO2 + 1 wt% La2O3
D. 99 wt% ZrO2 + 1 wt% Al2O3
Preparation Method Effects
The catalyst preparation methods can also affect the catalytic performance of the resulting catalysts. Aupretre et al.14 prepared a MgAl2O4 spinel support by solid-solid reaction between MgO and Al2O3. Compared with the Al2O3-supported catalysts, the spinel-supported catalysts exhibited a slightly higher basicity; whereas the surface acidity was strongly reduced. The MgAl2O4 was impregnated with different Rh precursor salts (nitrate, chloride, acetate) to obtain the supported Rh catalysts, which gave less acidic catalysts with greatly enhanced ethanol SR performances compared with the Rh catalysts supported on MgxNi1-xAl2O4/Al2O3. In this case, the Rh precursor salts had a substantial impact on the properties of the final catalysts. The use of a Rh nitrate precursor dramatically increased the overall acidity of the catalyst, while a Rh acetate precursor gave the catalyst with lowest acidity. Rh chloride resulted in catalysts with moderate acidity, allowing the preparation of well-dispersed Rh catalysts that were very active and stable for ethanol SR. Zhong et al.12 prepared ZrO2 supports using a co-precipitation method (ZrO2−CP) and a hydrothermal method (ZrO2−HT). A significant enhancement in catalytic activity, selectivity, and stability was achieved by the ZrO2−HT-supported Rh catalyst compared with its ZrO2−CPsupported counterpart at temperatures below 400 °C. Relatively strong Lewis acidic sites were found on the Rh/ZrO2−HT catalyst, which was responsible for the strong absorption of ethanol and the subsequent C−H cleavage. In contrast, on the Rh/ZrO2−CP catalyst, the ethanol adsorption was weak and the C−C cleavage was the dominating reaction. Recent studies15 have shown the effectiveness of the HT approach coupled with an optimized calcination treatment on the preparation of a nanosized, hydrothermally stable ZrO2 support of less than 20 nm (Figure 2). Pt deposited on this ZrO2 support showed enhanced activity for WGS reaction compared to a series of commercial ZrO2-supported Pt catalysts. This ZrO2 support may have great potential in bioethanol SR application.
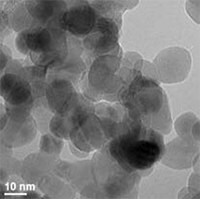
Figure 2.TEM image of ZrO2, ZrO2 was prepared using the HT synthesis method coupled with optimized calcination.
Promoter Effects
The introduction of alkali or alkali earth metal species into the supported Rh catalysts can improve the catalyst stability and performance. Roh et al.16 studied the deactivation of Rh/CeO2−ZrO2 catalysts for ethanol SR at 350 °C with a high space velocity of 480,000 cm3/g·h. Ethanol conversion decreased from 70% to 6% within 5 h due to coke deposition. The addition of 0.5% potassium increased the catalyst stability at the same reaction temperature of 350 °C. Chen et al.17 introduced Ca to neutralize the acidity of Al2O3, which helped decrease the undesired reaction pathway of the ethanol dehydration. Furthermore, the addition of Fe into this Ca−Al2O3-supported Rh catalyst allowed the transfer of CO from Rh to Fe species for subsequent WGS reaction to produce CO-free H2, which improved the Rh catalyst stability. Indeed, the acidic and basic properties of the supports strongly affect the SR reaction selectivity toward acetaldehyde and ethylene.14 Basic sites on the supports benefit the ethanol dehydrogenation step in acetaldehyde formation while the acidic sites need to be neutralized to decrease the ethylene formation.
Loading Effects
Catalyst loading also affects ethanol SR reaction activity. Roh et al.10 discovered that, for ethanol SR at 450 °C, the H2 yield based on per mole of ethanol decreased in the order: 2% Rh/ZrO2−CeO2 > 1% Rh/ZrO2− CeO2 > 3% Rh/ZrO2−CeO2, possibly due to differences in metal dispersion. Ethanol SR has been more extensively studied over the temperature range 600−700 °C since H2 selectivity is increased at these high temperatures. Liguras et al.18 reported the effect of metal loading on catalytic performance over Ru/γ−Al2O3 catalysts. They observed that the ethanol full conversion temperature was 825 °C for the 0.5% Rh catalyst, which was lowered to 775 °C in the case of the 2.0% Rh catalyst. Furthermore, the selectivities toward H2 and CO2 were substantially enhanced with increasing Rh loading. Additionally, the 2.0% Rh/Al2O3 catalyst fully converted ethanol without the formation of acetaldehyde or ethylene at temperatures close to 800 °C. The observed differences in catalytic performance were attributed to the increased number of active sites but not the differences in particle sizes with increasing metal loadings, since the rhodium particle sizes were ~2.1 nm for all these Rh/Al2O3 catalysts.
Conclusions
In the present review, an overview of previous ethanol steam reforming (SR) studies focusing on Rh has been given. Rh has been identified as the most active metal for ethanol SR reaction. Catalyst support, catalyst preparation method, metal additive promoter, and Rh loading all play important roles in optimizing performance of the ethanol SR reaction. The catalyst performance characteristics suggest a strong metal−support interaction. The ethanol SR reaction network is complex and water-gasshift (WGS) reaction also plays an important role in the overall reforming process. Undesirable reaction pathways such as ethanol dehydration and CH4 decomposition can lead to coke deposition and, consequently, deactivate the catalyst. The use of a binary support system such as MgO−Al2O3 and CeO2−ZrO2, and the introduction of hetero-metal species may inhibit carbon deposition leading to better Rh dispersion and, consequently, improve catalyst performance for clean H2 production from bioethanol. Finally, the introduction of new nanosized hydrothermally prepared catalyst support materials presents a promising avenue toward increased catalytic performance for ethanol SR.
Acknowledgment
This material is based upon work supported as part of the Institute for Atom-efficient Chemical Transformations (IACT), an Energy Frontier Research Center funded by the U.S. Department of Energy, Office of Science, Office of Basic Energy Sciences.
References
Pour continuer à lire, veuillez vous connecter à votre compte ou en créer un.
Vous n'avez pas de compte ?