Discovery and Single Crystal Growth of Lanthanide Intermetallics—Interplay of Synthesis and Physical Properties
Devin C. Schmitt, Michael J. Kangas, Julia Y. Chan
Louisiana State University, Department of Chemistry 232 Choppin Hall, Baton Rouge, LA 70803
Correlated Systems
Solid state and materials chemistry have made a tremendous impact and have experienced growth in recent years, particularly for rare earthcontaining materials. This is motivated by the potential applications impacting a vast number of areas in materials research. Materials containing f-orbital electrons are ubiquitous in technological applications involving electronic, magnetic, thermal, and optical properties. We have been able to exploit the self-flux growth techniques1,2 to grow single crystals over the last several years. Synthesis of solid-state materials by flux growth has made a significant impact in both fundamental and application-driven research and is part of the National Academy of Science grand challenge,3 specifically highly correlated materials, a class of materials with strong electron-electron interactions. A Physics Today article highlighted the need for high quality single crystals necessary for the discovery of novel materials with interesting properties.4 A good read is also “Fishing the Fermi Sea”, which describes the importance of making highly correlated materials in single crystal form.5
To understand the chemistry and physics of compounds with f-electrons, it is vital that materials be made in single crystal form so intrinsic properties can be studied. This involves methods such as self-flux growth, Czochralski, hydrothermal, and microwave methods. Our work has been motivated by the various functional classes of materials. In this review, we will highlight several classes of materials, including highly correlated and energy materials containing lanthanide elements—specifically synthesized by flux growth.
The focus of our research over the last several years has primarily been crystal growth of highly correlated intermetallics with Group 13-15 main group elements (Figure 1).6 We have discovered and worked on compounds that show heavy electron mass behavior (containing Ce) and compounds that exhibit unusual magnetism. While most materials are serendipitously discovered, systematic and rational methods must be developed.6
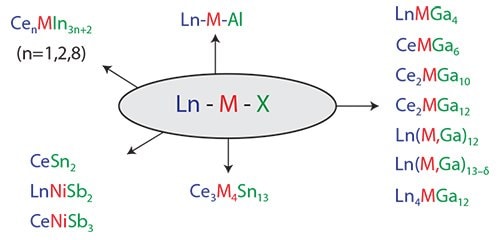
Figure 1.Representative lanthanide intermetallic materials illustrating the range of stoichiometries obtainable through molten metal flux growth. Lanthanide metals, transition metals, and flux elements are denoted in blue, red, and green, respectively.
Highly correlated systems have generated much interest, especially in the search for quantum materials, unconventional superconductors, and magnetic materials. Many of the compounds and systems discovered in this group are also highly field dependent, exhibiting large magnetoresistance, and showing unusual anisotropic magnetic behavior. While Ce-containing intermetallics have attracted much attention in the past, these unusual phenomena have also been extended to Pr- and Yb-based intermetallic phases. The skutterudites PrM4Pn12 (M = Fe, Ru, or Os; Pn = P, As, Sb), Pr(Cu,Ga)13, and PrAgIn2 have been studied because of diverse behavior such as superconductivity, unusual magnetic ground states and heavy electron behavior. Only with high quality single crystals can the origin of these properties be elucidated.
More recently, magnetization measurements of the ultrapure f-electron-based superconductor β-YbAlB4 7 demonstrate zero field quantum criticality without tuning external parameters such as high magnetic fields and pressures in a metal.8 These properties can only be determined with clean and high-quality materials.
Energy Applications
Lanthanide-containing compounds are not only interesting for fundamental science but can have direct implications in device-level applications. On the energy front, fields such as refrigeration and power generation have traditionally been dominated by mechanical phasechange technologies with relatively low efficiencies. A general drive in the materials and engineering community has been to replace these devices by thermoelectric and magnetocaloric solid-state materials with equal or higher efficiencies and much lower failure rates. Due to interesting magnetic properties and high atomic weight, lanthanidecontaining compounds, or rare earths, are excellent candidates for thermoelectric and magnetocaloric materials, respectively.
Thermoelectric Materials
Thermoelectrics are materials with the ability to convert thermal energy to electrical energy or use electrical energy to produce a temperature gradient. Their efficiency is measured by the dimensionless figure of merit ZT, which has components of electrical conductivity, Seebeck coefficient, and thermal conductivity. Characteristics of an ideal thermoelectric material include low thermal conductivity similar to a glass and high thermoelectric power factor (the product of electrical conductivity and Seebeck coefficient squared). To date, the best thermoelectric materials have ZT between 1 and 2, but a ZT ~4 is needed for competitive cooling and power generation.
YbAl3 is an example of the potential for rare earth-based intermetallic compounds for thermoelectric materials. YbAl3 has a thermoelectric power factor of 180 μW/K2-cm at 300 K due to Yb valence fluctuations; the highest known value for any thermoelectric material. However, due to very high thermal conductivity at room temperature, YbAl3 is not a suitable thermoelectric material.9 We can visualize this by considering thermal conductivity as a parasitic value. The higher the thermal conductivity, the more heat is allowed to leach through the system without contributing to voltage generation. In cooling applications, high thermal conductivity translates into a relatively small temperature gradient that can be established between the hot and cold sides of the device. If, however, we were able to keep the power factor of YbAl3 constant and reduce the thermal conductivity to as low as a glass (~1 W/m-K), we would find a ZT of 5.4 at room temperature— competitive with residential-grade refrigeration devices but with greatly increased longevity. Alternatively, as a power generating device, a ZT of 5.4 would correspond to >40% higher fuel efficiency in an automobile (using similar estimates to that of Bell).10
Rare earth-containing thermoelectrics not only have the benefit of potential valence instabilities, but they can form complex structures and have high atomic masses, both of which contribute to a significant reduction in thermal conductivity, which can improve performance as a thermoelectric. Substitution of the isovalent, but much higher atomic mass Yb for Ca in Ca14MnSb11 led to Yb14MnSb11.11 Yb14MnSb11 is a complex Zintl intermetallic material with a high thermoelectric power factor at elevated temperatures and a low room temperature lattice thermal conductivity of ~0.45 W/m-K, partly due to the high average atomic mass of the material. Optimized Yb14MnSb11 is the highest performing elevated temperature p-type thermoelectric material to date, with a ZT >1 at 1,200 K.12
Rare earths can be used to tune the electronic structure of a material. The Co4Sb12 skutterudite (Figure 2) is a small bandgap semiconductor with a large thermoelectric power factor. The thermal conductivity of Co4Sb12 is far too high for thermoelectric applications (>10 W/m-K), but the skutterudite structure contains voids large enough for rare earth atoms to reside. Rare earth ions act as both charge donors to modify electronic properties and phonon scattering centers to significantly reduce thermal conductivity. Iron can then be substituted for Co to return the compound to a semiconducting state. The filled skutterudite Ce(Co,Fe)4Sb12 has a large thermoelectric power factor, partly due to f- and d-electron hybridization, and a greatly reduced thermal conductivity, leading to ZT ~1 at room temperature.13
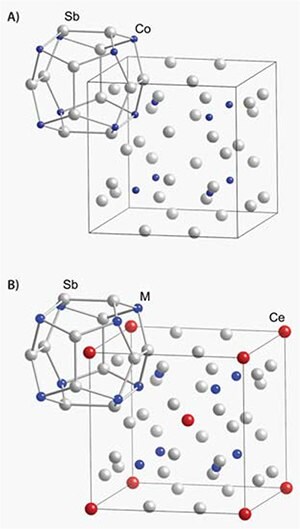
Figure 2.Crystal structures of the unfilled (A) and filled (B) skutterudite structure illustrating the filling of voids by lanthanide elements (example: Co4Sb12 and Ce(Co,Fe)4Sb12). Blue spheres represent the transition metal (Co or Fe). Grey and red spheres represent Sb and Ce, respectively.
The goal of a ZT >4 in order to provide competitive materials for cooling/power generation in bulk materials is not unrealistic, as Goldsmid proposes.14 This “limiting” factor was set using the best values of the parameters in ZT (ρ, S, and κ) found for bulk materials. Indeed, these values are conservative, and the estimation will likely increase as new thermoelectric materials are discovered. The high atomic masses and potential valence instabilities of rare earth containing intermetallic compounds make them ideal candidates for reaching the goal of ZT >4.
Magnetocaloric Materials
The magnetocaloric effect offers the possibility of an efficient and environmentally friendly refrigeration technology, and is based on utilizing the entropy changes (ΔSm) due to magnetic spins aligning in an applied field. This effect is caused by the coupling of the magnetic sublattice with the magnetic field, leading to the change in the magnetic part of the entropy of a solid. Cooling based on the magnetocaloric effect has already been demonstrated, but research is needed to find magnetocaloric materials with improved properties. An ideal magnetocaloric material should possess (1) a large magnetic moment so the entropy change will be large when the spins order and (2) a magnetic ordering temperature in the relevant temperature range. Other properties include high thermal conductivity, good mechanical properties, low cost and low toxicity. Rare earth compounds are excellent candidates because they not only possess large magnetic moments but can be easily optimized via substitution with other rare earth elements. Gd metal and its alloys show a large magnetocaloric effect near room temperature and are frequently used as benchmark.
A great number of binary, ternary, and quaternary rare earth-containing intermetallics have been investigated and reviewed recently.15,16 The materials range from being active at a few tens of Kelvin to above room temperature and exhibit many types of magnetic transitions. Magnetocaloric properties in Gd5Si4 and Gd5(SixGe1-x)4 alloy (Aldrich Prod. Nos. 693510, 693502) based materials have been reviewed.17 These compounds order ferromagnetically near room temperature. In these materials, the magnetic entropy change is enhanced due to the combination of the magnetic ordering at the desired temperature coinciding with a structure change.
La(Fe1-xSix)13 alloys have been shown to be effective magnetocaloric materials near room temperature. The magnetic properties can be tuned by doping both the Ln and Fe/Si sites, as well as through the incorporation of light elements into interstitial sites. In these compounds, the ordering temperature increases linearly with lattice expansion. Interestingly, in this structure type, the magnetic transition can be first order or second order depending on composition.18
Anisotropic properties are also important in magnetocaloric materials. In the oxide ErAlO3, orienting the crystal along the c axis more than doubles the magnetic entropy change compared to the a or b axes, with ΔSm of approximately -180, -70 and -80 mJ/cm3-K for a 2T change.19 In addition to optimizing the magnetocaloric effect by orienting single crystals along select crystal axes, changing the orientation of single crystals in a fixed magnetic field can exhibit the magnetocaloric effect. This effect is termed the anisotropic magnetocaloric effect. In DyNi2, the effect is slightly larger for a change from [001] - [110] in a constant field of 2 T than for a polycrystalline sample in a field change of 0-2 T, with ΔSm values of approximately -132 and -110 mJ/ cm3-K, respectively.20
Synthesis
The majority of samples characterized for magnetocaloric and thermoelectric applications are polycrystalline and have been synthesized through arc-melting or other high temperature methods. Arc-melting allows precision doping and tuning of magnetic and electronic properties by stoichiometric weighing of reactants. Polycrystalline samples contain many crystallites oriented in all directions, providing an average of the magnetocaloric effect and an overall reduction in thermal conductivity for thermoelectric applications due to boundary scattering. However, the growth of single crystals through flux growth or other techniques presents a number of advantages that include more complete structural characterization and determination of anisotropic magnetic and electrical properties. Single crystals can be oriented to the direction that maximizes the magnetic entropy change or the carrier mobility in anisotropic materials.
For example, we have grown high quality single crystals of CePdGa6, Ce2PdGa10, and Ce2PdGa12 using the self-flux method by reacting high purity Ce (Aldrich Prod. No. 263001) and Pd (Aldrich Prod. No. 203939) in excess Ga (Aldrich Prod. No. 203319) as a reactive flux in a 1:1:20 ratio. Elements are placed in an alumina crucible, sealed in an evacuated fused silica tube, then heated to a maximum dwell temperature of 1,423 K. They are then slowly cooled to the desired spin temperature, centrifuged, and subsequently etched to remove residual Ga flux. The reaction products, in this case, depend solely on the spin temperature selected (Figure 3). Larger crystals of CePdGa6 were grown using an alternate temperature profile and a reaction ratio of 2:3:30.6

Figure 3.Heating profiles for the synthesis of single crystalline Ce-Pd-Ga phases. Different spin temperatures yielded different stoichiometries, as indicated by varying spin/quench temperatures.
High quality single crystals of each ternary phase were characterized by single-crystal X-ray diffraction. As shown in Figure 4, each compound adopts a distinct tetragonal structure. CePdGa6, a heavy-fermion material, crystallizes in space group P4/mmm with a = b = 4.350(3) Å and c = 7.9230(5) Å. Ce2PdGa10 adopts a different tetragonal structure (I4/mmm) with lattice constants a = b = 4.3230(3) Å and c = 26.536(3) Å. Finally, Ce2PdGa12, an antiferromagnetic material, crystallizes in space group P4/nbm with lattice parameters a = b = 6.1040(2) Å and c = 15.5490(6) Å.
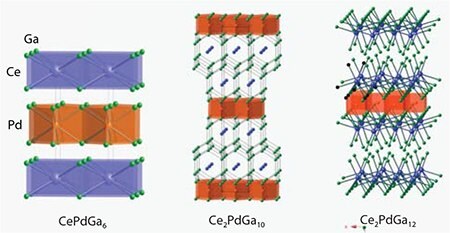
Figure 4.Crystal structures for CePdGa6, Ce2PdGa10, and Ce2PdGa12. Blue, orange, and green spheres represent Ce, Pd, and Ga atoms, respectively.
Starting stoichiometry also plays a key role in determining the structure of the final product, as seen in the case of Cu-containing lanthanide intermetallics. By changing the initial reaction ratio from 1:1:20 to 1:5:20, three different structure types are stabilized (Figure 5), yielding phases that exhibit properties ranging from heavy-fermion behavior to large positive magnetoresistance. By controlling both the reaction/spin temperatures as well as the starting stoichiometries, a wide range of possible structures are accessible through the metal flux technique.
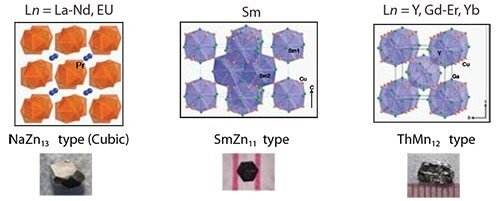
Figure 5.Changing the reaction ratio of Ln:Cu:flux to 1:5:20, three additional structure types are stabilized: NaZn13 type, SmZn11 type, and ThMn12 type (left to right).
Conclusions
The search is just beginning—the lanthanides are a truly functional toolbox for the discovery of novel materials with properties that are just yet to be discovered and understood.
Acknowledgments
The authors acknowledge NSF-DMR0237664, NSF-DMR0756281, and NSF-DMR1063735 for funding and the Chan Research Group for useful discussion.
References
To continue reading please sign in or create an account.
Don't Have An Account?