Ultra-High Efficiency Perovskite–Perovskite Tandem Solar Cells
Zhen Li and Kai Zhu
Chemistry and Nanoscience Center, National Renewable Energy Laboratory, Golden, Colorado 80401, USA
Material Matters, 2018, 13.1
Introduction
Next generation solar cells have the potential to achieve conversion efficiencies beyond the Shockley–Queisser (S–Q) limit while also significantly lowering production costs. To date, only multi-junction solar cells are able to achieve such high efficiencies. However, the current high-efficiency multi-junction cells require complex III-V-related epitaxial thin films and the use of an expensive single-crystal substrate, preventing low cost production of III-V multi-junction thin-film solar cells. In contrast to III-V films, polycrystalline thin-film solar cell technologies offer much lower production cost, but only moderate efficiencies. Fabricating highly efficient multi-junction polycrystalline thin-film solar cells remains a challenge.
Organic-inorganic halide perovskites have recently emerged as a new class of light absorbers for solar conversion applications. The power conversion efficiency of single-junction perovskite solar cells (PSCs) has already surpassed conventional polycrystalline thin-film solar cell technologies and is projected to compete with single-crystalline solar cells. Remarkably, these high-efficiency perovskite solar cells can be made from polycrystalline materials by either solution processing or vapor phase growth. Along with continued efforts to advance singlejunction PSC technology, many research groups are developing perovskite-based tandem devices in order to move beyond the S–Q limit. With recent material innovations in low-bandgap (1.1–1.3 eV) and wide-bandgap (1.7–1.9 eV) pervoskite absorbers and the transparent conductive interconnection layers, the use of polycrystalline halide perovskites for both the top and bottom cells in a tandem structure is a promising combination for the development of ultrahigh efficiency dual-junction thin-film tandem solar cells. In principle, such a device structure can be solution processed and promises to achieve efficiencies of >35% at one sun.
Here we review recent progress on perovskite-perovskite tandem solar cell development. In general, tandem solar cells can be constructed in two ways. In one approach, known as the fourterminal or 4-T tandem configuration, two independent solar cells are mechanically stacked one on top of the other. In the second configuration, referred to as two-terminal or 2-T tandem, the top and bottom cells are connected in series on a single substrate to form a monolithic device. Regardless of the exact tandem structures, bandgap engineering of perovskite absorbers is essential for achieving efficient perovskite tandem devices. In a tandem structure, the wide bandgap cell is on the top to harvest high-energy photons and to allow low-energy photons to pass through for harvesting by the low-bandgap bottom cell. For a 2-T tandem device, assuming infinite absorber thickness for top and bottom sub-cells, the best bandgap combinations are around 1.7 and 1.1 eV for the top and bottom absorbers, respectively; the best efficiency in theory is 38.7%, surpassing the S–Q limit of a single-junction device (33.7%).1 In actual devices, the thickness of the top cell can be adjusted to allow more photons to reach the bottom cell. Optical thinning can provide more material selection flexibility in tandem solar cells. For 4-T configurations, the current-matching restriction is removed and there is more freedom than in 2-T tandem solar cells to optimize the bandgap combination and gain higher efficiency.
Wide-Bandgap Perovskite Solar Cells
Wide-bandgap (~1.7–1.9 eV) perovskites are desirable for use as top sub-cells in tandem devices. Early stage development efforts centered on Br-I mixing of methlyammonium lead halide perovskites (MAPbX3). Noh et al. first demonstrated mixedhalide PSCs by replacing MAI with MABr in one-step precursor solutions.2 By adjusting the Br-I mixing ratio, the bandgap of MAPbI3–xBrx can be tuned from about 1.6 eV to 2.3 eV. Device performance also varies significantly with the Br-I mixing ratio. The open-circuit voltage (VOC) and fill factor (FF) both increase with moderate Br ratios (x<0.2), and the VOC decreases abruptly with a Br ratio higher than 0.2. The use of Br is also found to strongly increase perovskite stability against humidity.
Other studies also demonstrated the ability to tune the bandgap with bromine, but did not focus on the optimization of bandgap for tandem applications. Zhao et al. first studied the optimization of mixed-halide PSCs targeted for tandem solar cells.3 They reported a general additive-assisted one-step solution growth to prepare MAPbI2Br (Eg = 1.8 eV) thin films from a precursor containing the mixture of PbI2, MABr and MACl. The addition of MACl additive eliminates the formation of dendrite structures, and results in compact and uniform thin films of MAPbI2Br nanosheets. Planar PSCs with MAPbI2Br nanosheets achieved a power conversion efficiency (PCE) of 10%. Bi et al. used solvent-assisted thermal annealing (often referred to as solvent annealing) to grow MAPbI2.4Br0.6 perovskite thin films with an increased grain size.4 A small amount of DMF vapor used during thermal annealing increased the average grain size from about 300 nm to 1000 nm, resulting in a MAPbI2.4Br0.6 based wide bandgap device with a PCE of 13.0%.
Shortly after the successful demonstration of wide-bandgap MAPb(I1–xBrx)3 PSCs, Hoke et al. discovered the light-induced phase segregation phenomenon in I-Br mixed perovskites. It was found that once the Br ratio exceeds a threshold (~20%), I– and Br– ions start to segregate under light excitation, separated I and Br rich phases form. The phase segregation is recoverable after keeping the perovskite in the dark for a period of time. The light-induced low-bandgap phase acts as the carrier trapping defects and causing potential loss. The lower VOC is normally a limiting factor to achieving higher efficiency in wide-bandgap PSCs. Interestingly, the A-site alloying can affect the phase stability of Br-I mixed wide bandgap perovskites (Figure 1). For example, McMeekin and Snaith et al. reported better phase stability of FA0.83Cs0.17Pb(I0.6Br0.4)3.5 Doping FA+ with a suitable amount of Cs+ reduces the Goldschmidt tolerance factor in the alloy materials and stabilizes the perovskite cubic structures. The FA-Cs alloys also have a lower tendency for halide segregation under illumination; FA0.83Cs0.17Pb(I0.6Br0.4)3 PSCs with bandgaps of ~1.74 eV achieved a high VOC of 1.2 V and PCE over 17%. Rehman et al. further characterized the phase stability of the mixed-cation, mixed-halide CsyFA1-yPb(I0.6Br0.4)3 perovskite materials6 and found a strong correlation between phase stability and crystallinity of the materials. X-ray diffraction showed minimal full width at half maximum (FWHM) of the perovskite peak with 20% Cs+ (y = 0.2). Photoluminescence (PL) peak position of perovskite with 20% Cs+ remained constant after continuous illumination for 30 minutes. The samples with lower (5%) or higher (60%) Cs+ showed significant red-shifts in the PL spectra, indicating the formation of I-rich phase in the perovskites. In addition to better phase stability, samples with 20% Cs+ also showed the highest mobility and longest PL lifetime within the studied composition range (0 < y < 0.8). Using a similar perovskite composition, Yu et al. fabricated 1.75 eV wide bandgap PSC with PCE of 17.7%. Pb(SCN)2 additive and solvent annealing were used to improve the grain size and crystallinity of the perovskite absorber, thus enabling high efficiency.7 Lin and Huang et al. adapted a triple A cation composition (FA0.83MA0.17)0.95Cs0.05Pb(I0.6Br0.4)3 and fabricated p-i-n structure wide bandgap PSCs with PCE of 18.5%.8 The solar cell performance parameters and PCEs of several of, both mixed cation and anion, wide-bandgap PSCs are summarized in Table 1.
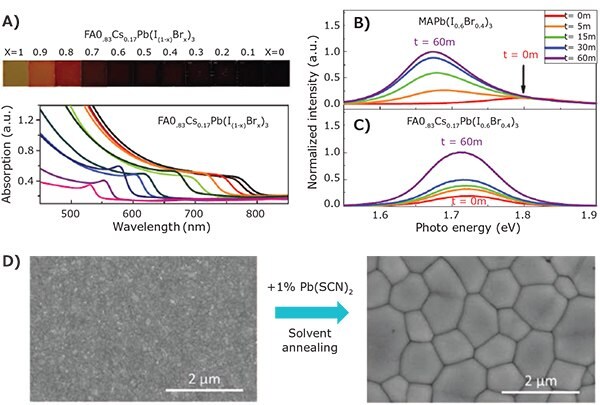
Figure 1. Wide bandgap perovskite materials. A) Optical images and UV-Vis spectra of FA0.83Cs0.17Pb(I(1-x)Brx)3 mixed halide perovskite with different Br ratios. B) PL downshifting from MAPb(I0.6Br0.4)3 indicating I-rich phase formation after light soaking. C) Stable PL emission from phase stabilized FA0.83Cs0.17Pb(I0.6Br0.4)3.5 D) Morphology evolution of FA0.83Cs
a: Stabilized power output (SPO) if available. b: SPO
Low-Bandgap Perovskite Solar Cells
Low-bandgap perovskite absorbers that can harvest photons in the infrared range are essential for constructing efficient perovskite-perovskite tandems. The most efficient way to reduce the bandgap of perovskite materials is by completely or partially replacing Pb2+ with Sn2+. The major challenge to developing Sn-based perovskites are the high carrier density and short carrier lifetime associated with the rapid oxidation of Sn2+ to Sn4+. Early studies focused on pure Sn (no Pb) based perovskites have fallen short on PCE and stability. Hao et al. used solution-processed lead-free MASnI3 perovskite as a light harvester in solar cells and attained a PCE of 5.23%.12 Incorporation of Br leads to an improvement in the PCE to 5.73% using a MASnIBr2 absorber. Noel et al. showed that the recombination rate in MASnI3 is extremely fast with a PL lifetime of ~200 ps and a high hole doping density (~1018 cm-3) based on terahertz conductivity measurement.13 Significant efforts have been devoted to reducing the carrier density by suppressing the oxidation of Sn2+. Kumar et al. discovered that doping with SnF2 can significantly suppress the formation of Sn4+ defects in the solution-processed CsSnI3 thin films.14 They found that including 20% SnF2 additive in CsSnI3 reduces the carrier density and film conductivity by 2 orders of magnitude. The same strategy was applied in FASnI3-based solar cells with a similar effect.15 Lee et al. later showed that adding SnF2 with pyrazine can further improve film quality by removing plate-like SnF2 segregation, leading to improved device performance.16
Recently, some of the most significant breakthroughs in lowbandgap perovskites have been reported by groups developing Sn–Pb alloy based absorbers in an inverted device structure (i.e., p-i-n planar heterojunction structure). Yang et al. demonstrated MA0.5FA0.5Pb0.75Sn0.75I3 based planar heterojunction solar cells with a bandgap of 1.33 eV and a PCE of 14.19%.17 Interestingly, these solar cells showed significantly improved stability, retaining 80% of the initial PCE after 12 days of storage in ambient air. Eperon et al. fabricated FA0.75Cs0.75Sn0.5Pb0.5I3 PSCs with a more suitable bandgap (1.2 eV) for tandem applications.18 The best solar cell yielded a PCE of 14.1%. Liao et al. also fabricated 1.2 eV PSCs with composition of (FASnI3)0.6(MAPbI3)0.4 (Figure 2).19 The solar cell showed an average PCE of 14.39% with a stabilized PCE of 14.8%. These authors further found the grain size of the perovskite thin film can be increased with the film thickness, improving the efficiency to 17.6% with a thicker perovskite layer (17.01% with certification).20 Perovskite absorbers with large grains and better crystallinity yielded a longer carrier lifetime and provided better cell performance. The solar cell performance parameters and PCEs of several, of, both mixed cation and anion, low-bandgap PSCs are summarized in Table 2. It is worth noting that several factors contributed concurrently to the rapid and exciting progress on the low-bandgap perovskite development: (1) The Sn–Pb alloys have lower bandgap and absorb in a wider range of the spectrum compared to pure Sn based perovskites; this is caused by the large bowing effect in bandgap composition correlations.21–22 (2) The oxidation tendency of Sn2+ is suppressed with alloying Pb2+ cations. (3) An inverted planar heterojunction structure does not need an oxidation process for the HTM, which alleviates the unnecessary reaction between oxidative HTM dopants and Sn2+ cations.

Figure 2.(FASnI3)0.6(MAPbI3)
Perovskite–Perovskite Tandem Solar Cells
The success of tuning the bandgap of perovskite materials over a wide range of the solar spectrum has enabled the development of perovskite–perovskite tandem solar cells. The tandem concept is not only attractive for obtaining ultra-high efficiency as with all other tandem cell configurations, it also can significantly reduce the fabrication cost of tandem solar cells because both sub-cells can be fabricated with solution processes. To date, all perovskite based tandem solar cells have been demonstrated with both 2-T and 4-T configurations from either solution processing or vapor phase deposition (Figure 3). Using a non-ideal combination of perovskite absorbers, Li et al. demonstrated a 4-T perovskite–perovskite tandem solar cell with 2.3 eV MAPbBr3/carbon nanotubes top cell and 1.55 eV MAPbI3 bottom cell.24 The total efficiency of this tandem device was only 9.5%, which is lower than the bottom MAPbI3 cell alone. Later in the same year, Heo et al. fabricated a series-connected 2-T MAPbBr3-MAPbI3 tandem solar cell using a lamination process.25 In order to laminate the top and bottom perovskite solar cells together, a thick (~2 mm) hole transporting material (e.g., PTAA or P3HT) was used. Although the device showed a promising high VOC of 2.25 V, the poor FF (~0.56) and inappropriate light absorption limits the tandem PCE to about 10.4%. The poor FF suggested the lamination process did not form a conformal and continuous contact between the top and bottom sub-cells. With the recent success fabricating stable Sn–Pb based low-bandgap perovskites, Yang et al. demonstrated an all-perovskite 4-T tandem solar cell with a total PCE of 19.08%. In this study, the top cell is unfortunately based on a 1.55 eV MAPbI3 absorber, which significantly overlaps in light absorption with the 1.33 eV bottom cell.17
To fully realize the potential of perovskite–perovskite based tandem solar cells, the bandgaps of the top and bottom subcells should be paired properly. Toward this end, Eperon et al. achieved a breakthrough on perovskite–perovskite tandem devices using ideally matched bandgaps.18 In this study, a top cell with a bandgap of ~1.8 eV was fabricated using FA0.83Cs0.17Pb(I0.5Br0.5)3. The mixing of FA and Cs dramatically improved the stability of perovskites even with the high Br ratio as previously discussed. The bottom cell used a new composition based on Sn–Pb alloyed perovskite with a low bandgap of about 1.2 eV. One challenge to fabricating perovskite-onperovskite tandem devices is avoiding damage to the bottom perovskite cell during deposition of the top perovskite cell. In an ideal situation, the solvent used to deposit the top cell stack should be orthogonal to the bottom cell stack. However, this is impossible at the moment since the standard perovskite compositions can only be dissolved in a few common solvents with sufficient solubility. To address this issue, the authors demonstrated that coating a 200 nm indium tin oxide (ITO) layer on top of the 1.8 eV bottom perovskite cell can effectively protect the underlying bottom cell from solvent damage. This compact ITO layer also serves as an effective recombination layer to electronically connect the top and bottom sub-cells. The 2-T tandem device with the ITO recombination layer achieved a remarkable stabilized PCE of 17.0% with a VOC of 1.66 V and JSC of 14.5 mA/cm2. They further optimized the bandgap of the top cell to construct a 4-T tandem cell by coupling a 1.6 eV FA0.83Cs0.17Pb(I0.83Br0.17)3 top cell with the 1.2 eV bottom cell with an total power conversion efficiency of 20.3%.
Another way to avoid damaging the bottom perovskite cell during tandem cell fabrication is to use vapor phase deposition to grow the top perovskite sub-cell. In a recent study, Forgács et al. presented efficient monolithic tandem solar cells based on two perovskite absorbers with different and complementary bandgaps: a wide bandgap perovskite with ~2 eV Cs0.15FA0.85Pb(I0.3Br0.7)3 for the front sub-cell and standard ~1.6 eV MAPbI3 for the rear sub-cell.26 To build this device stack, the wide bandgap perovskite layer is first deposited by solution processing and all the remaining materials needed for the tandem devices are deposited using thermal evaporation. Instead of using a transparent conducting oxide (TCO) based transparent interconnection scheme, a p-n junction based on doped organic semiconductors consisting of p-doped TaTm:F6- TCNNQ (40 nm)/n-doped C60:PhIm (40 nm) is used as an effective recombination layer to connect the two perovskite sub-cells. Despite the non-ideal bandgaps in the tandem device, a record efficiency of 18% was achieved for a monolithic 2-T tandem cell. The photovoltaic performance of all-perovskite tandem solar cells with different configurations are summarized in Table 3.
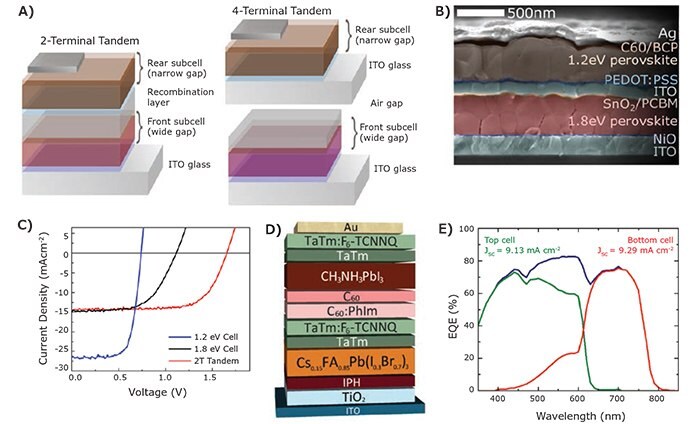
Figure 3.Perovskite–perovskite tandem solar cells. A) Schematics of 2-T and 4-T tandem solar cells. B) Cross-section SEM of a 2-T perovskite–perovskite tandem device. C) J-V curves of 2-T tandem cell.18 D) Schematics of a 2-T perovskite–perovskite tandem cell fabricated with vapor phase deposition. E) External quantum efficiency (EQE) of the top, bottom, and tandem cells.26 Reprinted with permission: A – C copyright 2016 AAAS; D – E copyright 2017 Wiely-VCH.
At present, the record efficiency of perovskite–perovskite 4-T tandem solar cell is 21.2% with a stabilized efficiency of 21.0% as demonstrated by Zhao et al.20 However, the wide bandgap PSC only used a ~1.6 eV FA0.3MA0.7PbI3 perovskite absorber. As a result, the wide gap cell only contributed about 3% efficiency to the tandem device. In this tandem configuration, the top cell has a regular n-i-p configuration of FTO/SnO2/C60-SAM/FA0.3MA0.7PbI3/ Spiro-MeTAD/MoOx(10 nm)/Au(8.5 nm)/MoOx(10 nm). The first 10 nm MoOx layer acts as both hole buffer layer and wetting layer for Au deposition. A MoOx wetting layer changes the growth mechanism and allows the deposition of ultrathin Au of 8.5 nm without the formation of isolated islands. The second MoOx layer is employed to form a dielectric-metal-dielectric stack to tune the light dispersion characteristics of the semi-transparent electrode, enhancing the total transparency of the top cell. It is worth noting the PCE of the best 4-T tandem cell is still partially limited by the performance of the top cell as the bandgap of perovskite absorber is not wide enough to optimally match with the bottom cell. Further PCE improvement is expected when efficient top cells with wider bandgaps (~1.7-1.9 eV) and better transparent conducting electrodes are developed.
Conclusions and Outlook
In summary, organic-inorganic halide perovskites have shown great potential for ultrahigh efficiency tandem thin-film solar cells. Several breakthroughs have been made recently on single-junction solar cells based on low-bandgap as well as wide-bandgap perovskite absorbers. These advancements along with the successful demonstration of transparent top contact and interconnection layers have enabled demonstration of 18% 2-T and 21% 4-T perovskite-perovskite tandem cells. These efficiencies were obtained despite non-ideal combinations of bandgaps of the two perovskite sub-cells. Although these studies present encouraging results indicating the perovskiteperovskite tandem approach is viable, they further identify the areas of research for further enhancing the performance of perovskite solar cells with a tandem configuration. Further studies and advances should be continuously made in the development of low-bandgap (~1.1–1.3 eV) and widebandgap (~1.7–1.9 eV) as well as the interconnection layer to electronically and optically couple the top and bottom perovskite sub-cells as follows:
- For low-bandgap perovskites, research should be focused on increasing carrier lifetime and reducing defect densities for Sn-Pb based perovskites. The EQE in the near-IR wavelength range (about 700 to 900 nm and beyond) is currently not ideal. Increasing charge transport length without significant recombination loss is expected to increase EQE in the long wavelength. Reducing charge recombination (at interface, bulk, and/or grain boundary) can compensate to some degree for the loss of photocurrent when monolithically stacking two subcells. Moreover, reducing perovskite process temperature can help avoid degradation of the underlying sub-cells.
- For wide-bandgap perovskites, the development of ~1.7 eV bandgap materials has been quite successful in recent years. Thus, more effort should be directed toward a bandgap range of about 1.8–1.9 eV to allow more flexibility to pair top and bottom subcells. The voltage loss relative to the bandgap is still quite large for wide-bandgap PSCs; significant effort is required to reduce the VOC deficit to improve the power output of tandem devices.
- An effective interconnection layer is a transparent electrode with both good transparency (>90 transmission) and electrical conductivity (10–100 Ω/sq). Energy-level matching, robust adhesion and good chemical stability are key considerations of the transparent electrodes. In addition, this multifunction layer also needs to be robust enough to avoid damaging the underlying perovskite cell during deposition of the top perovskite cell stack. With advances in these areas, it is expected that perovskite-based tandem solar cells will be realized in the near future with efficiencies approaching or exceeding the S-Q limit.
Acknowledgements
We acknowledge the support by the hybrid perovskite solar cell program of the National Center for Photovoltaics funded by the U.S. Department of Energy, Office of Energy Efficiency and Renewable Energy, Solar Energy Technologies Office. The work at the National Renewable Energy Laboratory is supported by the U.S. Department of Energy under Contract No. DE-AC36-08-GO28308.
References
Pour continuer à lire, veuillez vous connecter à votre compte ou en créer un.
Vous n'avez pas de compte ?