Conducting Bio-based Polymers and Composites for Advanced Applications
Roger Gonçalves1,3, Marco A. B. Ferreira1, Ernesto C. Pereira1,2,3
1Center for Innovation on New Energies, Institute of Chemistry, Campinas State University, Campinas – SP, Brazil, 2Center of Excellence for Research in Sustainable Chemistry, Department of Chemistry, Federal University of São Carlos, São Carlos – SP, Brazil, 3Center for Research and Development of Functional Materials, Department of Chemistry, Federal University of São Carlos, São Carlos – SP, Brazil
Introduction
Climate change has become a harsh reality that cannot be ignored. To hinder its destructive impact, it is imperative to move towards a clean energy matrix with low dependence on fossil fuels and decrease the number of residues dispersed in the environment. Bio-based polymers can be essential in reducing electrical and electronic waste and increasing service life for targeted applications. This mini-review discusses the recent achievements in bio-based polymer synthesis, their properties, and their applications as composites.
Requiring renewable and greener pathways, the accelerating need for energy combined with the current call for sustainable growth is one of the most critical challenges in contemporary societies. Biomass is becoming a viable alternative or complementary source for obtaining petroleum-derived products, such as fuels, polymers, and fine chemicals. In this mini-view, we discuss the recent achievements in conductive bio-based polymers and their applications in bioelectronics, sensors, and supercapacitors.
Functional Materials for Greener Composites
The synthesis of sustainable materials for renewable energy generation has been the focus of substantial research in recent years.1 The fundamental challenges are long cycle life, high functionality, high performance, low cost, and biodegradability.
Materials such as carbon composites, hybrid materials, and engineered polymers can be optimized to satisfy these five criteria. Among these emerging resources, pure and composite bio-based polymers are promising to build cleaner and sustainable energy devices. In addition, the appeal for greener technological solutions encourages scientists to seek inspiration in nature itself, stimulating a new genre of research focused on the development of bio-inspired materials.2
Bio-based Polymers
Biopolymers, illustrated in Figure 1, are produced by living beings or obtained from renewable sources of raw materials, making them a greener alternative to conventional polymers. One great advantage of using biopolymers is their biodegradability in the environment. The degradation of these macromolecules results from the action of naturally occurring microorganisms.1,2 Therefore, biopolymers are gaining traction due to these characteristics increasing their useful life cycle and disposability. “Greener polymers”, such as “green” polyethylene (PE), are also widely employed. Greener PE has the same applications as fossil-based PE, with the extra advantage of capturing CO2 from the atmosphere during its production.3

Figure 1.Example of biopolymers sourced from either animal or plant. Reprinted with permission from reference 3, copyright 2018, Elsevier.
Biopolymers have also been investigated to replace the conventional active metals and alloys used in smart devices for increased energy saving.3 Active materials respond to external stimuli such as pH, temperature, humidity, and electricity by changing its shape, color, and/or size. This technological application trend occurs due to the intrinsic properties of polymers, such as low density, low cost, fracture resistance, malleability, easy processability, and fabrication.4
While several active polymers respond to electrical stimuli,1 biopolymers are nonelectrical conductor materials. Thus, electrically conductive fillers dispersed in the biopolymer matrix have been investigated.5 The filler dispersion in the matrix determines the percolation pathway for an electrically conductivity composite. Discussed next are the different dispersion techniques used to prepare conductive composites.
Preparation of Bio-based Composites
Purely physical mixing techniques such as high-speed mixing, melting mixing, and roll milling are conventional procedures to synthesize polymer composites. Each of these methods relies on shear forces to cause a filler to disperse in a polymer matrix. However, under different conditions, these processes could produce a lower-quality material. These techniques do not permit the addition of compatibilizers that improve dispersion, requiring the fillers to be pre-emptively modified.
Another approach is the use of chemical processes to improve dispersion. Among them are such techniques as chemical vapor deposition and in situ polymerization. While these processes are efficient, they require additional steps and have an increased cost for industrial scale-up. However, they also allow for the addition of compatibilizers without the need to modify both matrix and/or fillers.
One innovative approach is the Spatial Confining Forced Network Assembly (SCFNA) technique which allows for great conductivity using minimum filler. The electrical conductivity of short polypropylene/carbon fibers prepared using this method is up to four orders of magnitude above those produced using common incorporation technology.2 Also worthy of mention is a self-assembling technique involving the use of a poly-cation and a poly-anion in which electrostatic interaction enables the formation of a film. The low-cost point of this procedure and the possibility of preparing polycation/polyanion films comprised of upwards of a hundred layers make it an attractive method.6
Figure 2 schematically represents the several types of methods used to disperse conductive charges in polymer matrices.

Figure 2.Illustrative scheme of different techniques of dispersion of conductive fillers in polymeric matrices. Partially reprinted under Creative Commons Attribution License.
High-performance Bio-based Composites
Plant and bacteria celluloses are excellent examples of easily produced biopolymers from renewable sources. The following subsection will present the most recent advances in biopolymer applications.
Farjana et al.7 obtained a flexible sensor from bacterial cellulose and a solution containing 1 mg of carbon nanotubes (CNT) (Cat. Nos. 901019, 900788, 755168) per mL of cellulose. The conductive composite (up to 1.6 mS cm-1), showed excellent mechanical properties and a good electrical response to deformation. In a different publication, Sen et al.8 used cellulose and graphene (Cat. No. 900561) nanocomposite to obtain a composite with electrical conductivity of 5.1 mS/cm using only 0.50 wt.% graphene filler. Using another approach, Peng et al.9 fabricated graphene-cellulose nanocomposite films by casting using imidazolium-based ionic liquids (Cat. No. 900771). These films showed conductivities of up to 10 mS/cm starting from 200 mL of a solution containing 80 mg of rGO and 30 g of [BMIM]Cl. Also using ionic liquids, Javed et al.10 obtained nanofibers by electrospinning based on cellulose acetate (Cat. Nos. 419028, 180955), graphene oxide (GO, Cat. No. 921556) and 1-butyl-3-methylimidazolium chloride ([BMIM]Cl, Cat. No. 94128). The combined advantages of GO and [BMIM]Cl materials allow for the homogeneous dispersion of GO and better solubility of cellulose, demonstrating the compatibility effect these ionic liquids confer on polymeric matrices.11 A concentration of only 0.43 wt.% of graphene oxide was enough to give a conductivity of 5.30 mS/cm.
As previously stated, the electrical conductivity of biopolymers is extremely low, while synthetic polymers, such as polyaniline (PAni, Cat. No. 912891), poly(3,4-ethylenedioxythiophene) (PEDOT), polypyrrole (PPy, Cat. No. 912573), and polythiophene (PTh) have high electrical conductivity (Figure 3). While these polymers show good biocompatibility, their low degradability is a critical detractor for their use. A strategy to improve this proclivity is combining the synthetic with biopolymers, obtaining conductive compounds with strong biodegradability and excellent biocompatibility. The challenge in synthesizing these composites is finding the best composition to maximize electrical conductivity and minimize the proportion of non-degradable conjugate components. Another approach, in line with the precept of bioinspiration, is the use of conventional molecules, such as carotene derivatives (Figure 5A), present in carrots, to produce conductive composites based on a single molecule.12 Although the performance is not yet comparable to that of conductive macromolecules, it is a very promising field of research.
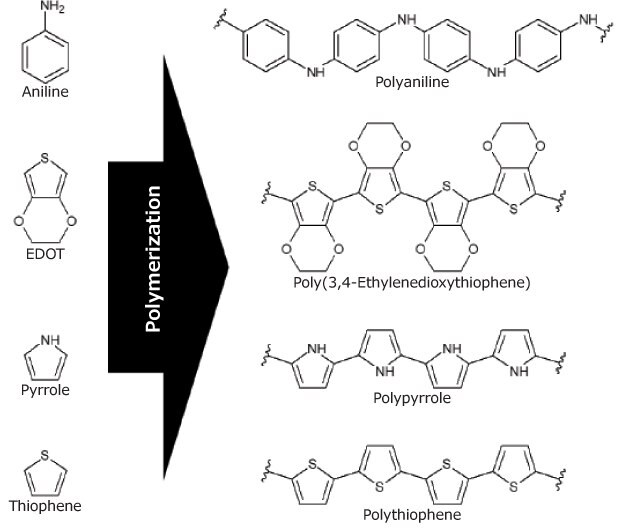
Figure 3.Chemical structure of the main monomers and their respective conducting polymers.
PAni has attracted significant attention due to its high electrical conductivity, easy synthesis in aqueous media, excellent thermal and environmental stability, controllable electrical conductivity, and ease of structural modification. This polymer has promising future applications in flexible electronics, such as electrodes and strain sensors.13 To use a biodegradable matrix and take advantage of the conductive properties of polyaniline, Han et al.,14 studied the in-situ polymerization of aniline in a nano cellulose (Cat. No. 561126) template to incorporate it into a natural rubber matrix (Figure 4). Aniline was integrated into the rubber via cellulose nanofiber (CNF) polymerization and complexation ensuring a better dispersion of the conductive portion and resulting in a homogeneous and flexible conductive composite (up to 90 mSm-1). The material was characterized as a strain sensor and supercapacitor, demonstrating excellent performance for both applications.
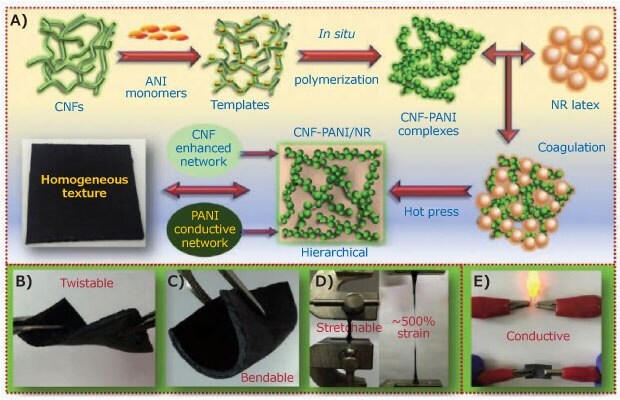
Figure 4.A) Schematic illustration for the preparation and synthesis process of conductive CNF-PANI/NR hybrid elastomers with a hierarchical 3D network structure and the demonstration of their B) flexibility, C) bendability, D) stretchability, and E) conductivity. Reprinted with permission from reference 14, copyright 2019 Elsevier.
PPy was among the first conductive polymers studied and has been widely used in bioelectronics and biosensors. It is prepared by the electrochemical or chemical oxidation of pyrrole. Different dopants can be inserted during its oxidation, affecting its conductive properties. An example of this phenomenon is how the introduction of poly(L-glutamic acid) as a dopant in PPy (Figure 5B) provides acid groups that further improve PPy’s electrical conductivity and biodegradability.15 Additionally, polypyrrole-block-poly(caprolactone) (Cat. No. 735817) is another biodegradable conducting polymer.
PEDOT is a conductive polymer based on the monomer 3,4-ethylenedioxythiophene (EDOT). PEDOT nanotubes have been successfully used for neural recording, closely mimicking the abilities of neurons.16 Additionally, the poly(3,4-ethylenedioxythiophene)-poly(styrene sulfonate) (PEDOT:PSS, Cat. No. 900208) composite is in regular use due to its unique properties, such as excellent conductivity, good stability, high optical transparency, and low toxicity. Therefore, due to the need for the presence of a second chain to improve the properties of PEDOT, using lignin (Cat. No. 370959) as a biopolymer (Figure 5C) has also conferred greater biodegradability to the composites of this conductive polymer without any damage to its conductive property, as lignin also acts as a dopant molecule and is successfully used as supercapacitor material.17
To improve poor mechanical properties, hydrophobicity, and non-degradability of these intrinsically conductive polymers, blends with biopolymers have been explored and extensively researched.1 Some successful biopolymers for these mixtures include polylactide (PLA, Cat. Nos. 764620, 764612, 767344), polycaprolactone (PCL, Cat. Nos. 440744, 440752, 704105), poly(lactide-co-glycolide) (PLGA, Cat. Nos. 901021, 790214, 900316, 900289), polycaprolactone fumarate, poly(lactide-co-polycaprolactone) (PLA-co-PCL, Cat. Nos. 906840, 900321, 900300, 900312), polyurethane (Cat. No. 446084), chitosan (Cat. Nos. C3646, 900342, 900341), gelatin (Cat. No. 1.04078), collagen (Cat. No. 234149), and heparin (Cat. No. Y0001282). It is important to mention that the application of biopolymers to produce biodegradable composites has been subject to well-established testing methods, such as ISO 14855-2, which regulates the durability properties of these materials in various simulated weather conditions.18
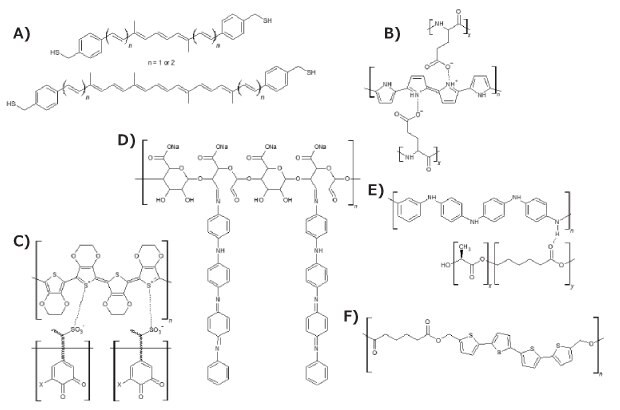
Figure 5.Chemical structure of bio-composites based on conductive polymers: A) carotene analogues, B) Ppy doped with poly(L-glutaminic acid), C) PEDOT:lignin, D) oligo PAni-graphitized polysaccharide, E) polyaniline/poly[(L-lactide)-co-(ε-caprolactone)], and F) 5'''-bis(hydroxymethyl)-3,3'''-dimethyl-2,2':5',2'':5'',2''-quaterthiophene-co-adipic acid polyester.
Wang et al.19 produced a polymer based on a polysaccharide backbone graphitized with polyaniline tetramers (Figure 5D) that is water soluble, biodegradable, electroactive, and noncytotoxic. Additionally, this innovative polymer exhibited electroactivity, reversible redox capabilities, and reversible doping/de-doping properties, proving to be an excellent candidate for medical applications, such as biosensors, active drug delivery, and nerve probes. Thus, regarding biomedical application, a polyaniline (Cat. No. 912891) enhanced poly[(L-lactide)-co-(ε-caprolactone)] copolymer (Cat. No. 769851) developed by Bhang et al.20 (Figure 5E) was used for the control of neural cell function. The fibers of this polymer were synthesized by electrospinning and then placed in PC12 cell culture medium, obtaining a porous material with a regular structure and with great neuro-regenerative capacity. To guarantee biodegradability and obtain electrical conductivity, Guimard and collaborators21 studied the incorporation of thiophene oligomers (Cat. No. T31801) in natural polyester, obtaining a conductive bio co-polymer. By means of a simple polycondensation reaction, alternating electroactive quaterthiophene units and biodegradable ester units were copolymerized resulting in the novel polymer 5,5'''-bis(hydroxymethyl)-3,3'''-dimethyl-2,2':5',2'':5'',2''-quaterthiophene-co-adipic acid polyester (QAPE) (Figure 5F). This material exhibited redox activity via cyclic voltammetry as well as red-shifted absorption peaks upon doping, confirming that the quaterthiophene units maintain their electroactivity. These examples demonstrate how wide and promising the use of bio-based composites for different applications can be, especially in greener and clean energy processes and devices.
Table 1 summarizes the essential biobased composites covered in the review along with their primary application and electrical conductivity magnitude, the most critical property for this type of material.
Final Considerations and Future Challenges
Although the development of conductive bio-based polymers still has a substantial way to go before replacing conventional polymer composites, they exhibit significant “green” potential in a world where sustainability is in focus. That said, critical improvements are still necessary for this vision to be realized. In particular, the fillers currently used to increase the conductivity of these composites are neither biodegradable nor “green”, and ongoing effort is still required to discover additives that match the low environmental impact of the base material. Fortunately, research investigating the production of more sustainable conductive loads has attracted considerable attention and important work has been done — much like in the case of graphene produced from biomass.22 In these possibilities, there is great room for optimism.
Acknowledgments
The authors acknowledge the financial support provided by GlaxoSmithKline, Brazilian National Council for Scientific and Technological Development — CNPq, São Paulo Research Foundation (grants no. 2013/07296-2, 2014/50249-8, 2017/11986-5), and the Coordenação de Aperfeiçoamento de Pessoal de Nível Superior (CAPES – Finance Code 001).
References
To continue reading please sign in or create an account.
Don't Have An Account?